Flash and JavaScript are required for this feature.
Download the track from iTunes U or the Internet Archive.
Description: This lecture covers the autonomic nervous system as well as the differentiation of the brain vesicles and introduces the hindbrain and segmentation.
Instructor: Gerard E. Schneider
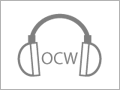
Lec 9: Autonomic nervous sy...
The following content is provided under a Creative Commons license. Your support will help MIT OpenCourseWare continue to offer high-quality educational resources for free. To make a donation or view additional materials from hundreds of MIT courses, visit MIT OpenCourseWare at ocw.mit.edu.
PROFESSOR: This is the ninth session, but we have a little bit from the last session to finish. I want to say just a little more about the autonomic nervous system. And this is where we ended last time. And let's go back to when we talked about the early development of the spinal cord and the formation of the sympathetic ganglia.
This was the picture we used. You see the thickening of the neural plate there, formation of the neural groove, and then the neural tube, formation of the dorsal root ganglia. But then, from those neural crest cells, we also have the formation-- some of these cells migrate and form the paravertibral ganglia, a series of interconnected ganglia alongside, just in front and to the side of the spinal cord. They're outside the vertebrae. In between the paravertibral ganglia and the neural tube there are the spinal vertebrae.
And then there's also the prevertibral ganglia. One is the largest, cilia ganglion, innervating the gut. So we're going to talk about that innervation, sympathetic innervation pattern, first. The preganglionic motor neurons that innervate these three ganglia you see hear, sympathetic ganglia, are found from the first thoracic segment in the cord to about the second lumbar segment. And if you look at this series of sections that we saw before in slightly different colors, you'll see the little lateral horn in these three segments. This is L1 and to of the thoracic segments. There you see the little protrusion of the lateral horn.
And if we take just a section-- this would be one of these sections-- there's the lateral horn, and I'm showing some of the preganglionic motor neurons. I will also show an axon descending from the brain that's connecting there directly to the lateral horn neurons.
So these are preganglionic of the sympathetic system. So they're sending their axon out to a ganglia, either the paravertibral ganglion here at that level or a prevertibral ganglion, like this cilia ganglion, which I show here sending an axon to the smooth muscle of the gut.
And notice that axons of the paravertibral ganglion cells send their axon back into the ventral root. These are the communicating nerves that connect the paravertibral ganglia, each of them, to the ventral roots. So the axon that goes back into the ventral root, besides to some of the intestinal tract, it's going to smooth muscles, glands, blood vessels, the arrector pili muscles, the muscles that make the hair fluff up, and sweat glands. And the upper thoracic, some of those nerves go to the cardiac muscles.
This is a typical textbook illustration. This is one, I think, from the earlier Brodal volume. This is Per Brodal. And it shows the spinal cord. These would be lateral horn neurons sending their axons out to terminate. The paravertibral ganglia-- he just shows the chain of ganglia on one side.
And then he shows a prevertibral ganglion here. There's also plexi. A plexus is just a tangle of nerve fibers. It may or may not have ganglion cells in it. The cilia ganglion, like you see here, has cells of the synthetic nervous system in it. And the axons of those cells then go out to the end organs.
Now, for the parasympathetic, it's quite different in the nature of the innervation. Because now the preganglionic motor neurons are up in the brain. They're in the dorsal motor nucleus of the vagus nerve. They're in salivatory nuclei, very tiny nuclei in the upper hindbrain, the rostral hindbrain, or in a little part of the third nerve nucleus, the Edinger-Westphal nucleus, the nucleus that controls the pupus.
OK, so now in the case of a parasympathetic system, the postsynaptic cell here, it gets the synapses from the brain, the axon coming from the brain. It's always near the end organ. So for the eye, it's just a ganglion behind the eye. And the axons go to the iris.
With the gut, it's cells right in the wall of the gut. And as we will see, they form an entire nervous system by themselves. But there you see the axon of the postganglionic cell innervating the smooth muscle of the gut. And the same is true for pelvic organs here.
This is, you can see, another way textbooks sometimes illustrate it here. They're showing three sections of the brain, midbrain, and two of the hindbrain, showing how the axons of the parasympathetic system go out to a ganglion. And they're just sketching some of those ganglia that are near the organs being innervated. And then you have the same thing with the [INAUDIBLE] for the innervation of the organs of lumenation and the sexual organs.
OK. So what about the neurotransmitters? How are the neurotransmitters different? Remember when we encountered this before? We talked about the discovery of Otto Loewi? What was his discovery? What was he studying the innervation of? The heart, exactly. And he found that it was a chemical released by the neurons that was causing the heart to speed up or slow down when the accelerator nerve or the decelerator nerve were activated.
But Loewi didn't actually discovered the neurotransmitters of the autonomic innervation of the heart. What are the neurotransmitters? Acetylcholine for both. It actually is true for both if we're talking about the neurotransmitter release in the autonomic ganglia. It's always acetylcholine-- sympathetic and parasympathetic.
But when the organ itself is innervated by the ganglion cell axon, then the neurotransmitters are different. So then what you were about to say is correct, they are different. Acetylcholine for the parasympathetic system in almost all cases and noradrenaline, or norepinephrine is the technical name, for-- that's the one that speeds the heart, norepinephrine.
Norepinephrine or noradrenaline is very similar. Adrenaline is actually a trade name, but it's often used. The molecule is very similar to that of adrenaline, released by the adrenal glands. And they have similar effects on the postsynaptic side. So here I've just shown the innervation of the heart and the innervation of the gut.
If you look at the gut here, I show acetylcholine being released in the cilia ganglion. And then the axon that innervates the gut is releasing norepinephrine, or is often just N-E in my abbreviations, or A-C-H for acetylcholine if it's parasympathetic.
And notice the preganglionic cell for the-- you could be way down in the lower gut. But the parasympathetic axon originates here in the dorsal motor nucleus, the vagus nerve in the hindbrain. So the reason it's called the vagus nerve, it's a wandering nerve. It goes through a lot of the body, from all the way up here to all the way down to the body. So vagus means the wanderer, the vagus nerve, the 10th cranial nerve.
So what about the enteric nervous system? I just mentioned it. Why do you think it's considered to be a separate system? It's it just the ganglionic neurons of the parasympathetic?
It actually isn't quite that way. It is that, but it's much more. First of all, it's semi-autonomous. That means the neurons connect with each other and interact. Peristalsis in the gut is regulated by it. That will go on even if the gut loses its innervation in the brain. And there may be as many neurons in that system as in the entire spinal cord.
This was all discovered in the last 20 years with advances in neuroanatomical methods. So most of these neurons are in the walls of the intestine. It's a network of multiple plexi. And here I list the different layers of the plexus, each containing neurons.
It's innervated by the vagus nerve. It's similar to the cardiac ganglion, but we think the cardiac ganglion also has some interconnections within itself. So maybe the heart has a brain too, but the heart definitely has, and it's called the enteric nervous system.
And I also point out here that various neurotransmitters are used in that system, various peptides, various neurotransmitters, not only acetylcholine. So that was a major discovery, very important in modern medicine, and of course opened up all kinds of new treatments with greater knowledge of the neurotransmitters there.
I want to discuss just briefly the hierarchy of central control in the autonomic nervous system. And the best way to illustrate that is to talk about control of body temperature. We know body temperature is regulated by autonomic nervous system. And you could say in general for autonomic system, there's different levels of control, spinal level, hindbrain level, midbrain level, and forebrain level.
Temperature regulation is the thing that's been studied most thoroughly by this woman, Evelyn Satinoff. She's written some very nice review papers. I think I cite a major review she wrote in the book as a suggested reading.
So what does this mean, that there's these multiple levels? It means that if you have an animal in which the spinal cord has been disconnected from the brain-- so the brain can influence only the head region, no longer influences the body-- you still have temperature regulation of the body. But it's not nearly as good as the temperature regulation is if the animal is intact.
If you leave the hindbrain connected but not the midbrain and the forebrain, then the regulation improves. But it's still not normal. The midbrain adds a little more, but only if you have the hypothalamus in the diencephalon, the tweenbrain do you get really normal endothermic regulation in warmblooded animals. Of course, all of this would be quite different if we're dealing with the poikilothermic animals, the animals that lose body temperature as regulated only by their behavior and not by internal mechanisms. And I'm not going to go through the supplementary figures.
So now, let's get into the hindbrain. And we'll talk a little bit about segmentation. Oh, wait a minute. Before that, I want to have that little intermission in the book, where it's important to talk at some point about the meninges and the glial cells. So let's just do that first here.
Remember how the whole nervous system forms around the ventricle. It's the walls of the tube. The cerebral spinal fluid that is inside that ventricle is pretty important because it has nutrients that it bathes with all the cells, especially the ones near the ventricle.
It's important for that. It's important in regulation of fluid balance. There are very specific brain regions involved in that. We'll be talking about that.
It's also a communication medium. This has not been studied all that much, but there are neurons that secrete substances into the cerebrospinal fluid. And because that fluid then [INAUDIBLE] through the ventricular system and through the penetration of that fluid into the intercellular space in the brain, there could be changes in the brain, because there are some regions where even fairly large molecules can get into the brain in that fluid.
So let's take a look at how that fluid is made. These are questions we want to answer. What cells make the cerebrospinal fluid? Originally it was just the fluid, the embryonic fluid. And then the neural tube closed, so now it's all inside. So the only way you're going to replenish that fluid now is if you have cells secreting it. But you have to keep in mind the cerebrospinal fluid isn't just in the ventricles. It's also outside the brain. How does it get out? It's around the outside, outside the pia.
We say it's in the subarachnoid space surrounding the brain, so if you wanted to see what that means and how it gets there. And then a term for a part of the ventricle is the aqueduct of Sylvius. We'll define that too.
First of all, can somebody tell me what cells make it? What are the choroid plexus cells? That's exactly right. What are they? They're ependymal cells. What are ependymal cells? They're cells that line the ventricle. The ependymal cells are those cells at the very inner edge of the central nervous system.
So in this picture, I show here places where that lining, the ependymal cells, which are all along the ventrical here. When the wall of the neural tube is thin there, some of those cells become-- they proliferate. So they proliferate so much that when you're doing a dissection in the adult brain and you enter the ventricle, you'll sometimes find them as clumps of red stuff. You don't realize what they are.
But here's what they are. They're these cells that have proliferated. So the length of this membrane has gotten much longer, and it sort of corrugates like that.
They are secreting cerebrospinal fluid into the lateral ventricles. And they secrete so much that it flows out of the lateral ventricles, as you see here with the arrows, and into the third ventricle, where there is more choroid plexus at the roof. But the third ventricle is a narrow ventricle that goes all the way down and all the way to the top. So there's not very much choroid plexus there. Most of the fluid is made here in the lateral ventricles. And it flows then caudally through this narrowed ventricle in the midbrain, where it gets a special name, the aqueduct of Sylvius, because Sylvius described it.
And then, when it gets to the fourth ventricle, which is much bigger-- remember the fourth ventricle widens out-- there are little openings to the outside, lateral apertures and one midline aperture. The lateral apertures, for those history buffs, are named for Luschka, because Luschka named them-- sorry, discovered them. And other people named it after him. And then there's one right on the midline that's named after [INAUDIBLE], because he described them.
So that's how the fluid gets out. Now, it also goes all the way down. It forms the cerebrospinal fluid of the cord also.
AUDIENCE: [INAUDIBLE].
PROFESSOR: There are openings. It flows right out into the subarachnoid space. So let's look at that, where it's flowing to. This is just what I said.
So now I want the layers of the meninges that surround the brain and spinal cord. And then I want a little more detail. I want to know what the pial-glial membrane is. What are the cells that make it?
But first of all, you should all know the meninges. Dura, which means tough mother, right. Dura mater-- it's not matter. It's not dura matter, it's dura mater, the tough mother.
And then the innermost one, what's that? The soft mother, right. Pia mother, the gentle mother. It's very thin, it's transparent. When you do a brain dissection, people will say, oh, make sure you remove all the meninges.
Make sure you remove the pia. How can you remove the pia? You can't even see it. It's so thin. It's people that don't know they're anatomy very well that ask you to do that. What they're really asking you to do is to pull out the arachnoid.
The arachnoid, what is the word arachnoid mean? Spider. It's like a spider's web. So I love this figure from Brodal. I made a new version of it for the book. He shows a little piece of it here and a little piece of the brain all the way down to the ventrical underneath.
So at the top you have the thick one. It's like canvas in monkeys and humans. In the rat, it's pretty thin. You see right through it. You don't see it very readily. But when you do surgery, you become aware of it, because it is tougher than the other layers. That's the dura.
And right below it is the cells of the arachnoid tissue. And notice that the arachnoid cells have extensions that go right down and attach to the pia. It's those attachments that make it look like a spider. And the arachnoid goes right down in between the hemispheres, for example, where the dura does not. There is dura between the hemispheres and the cerebellum. It goes in there and makes dissections difficult, because the dura is so tough.
And note here, the blood vessel is encased by those cells of the pia mater, the gentle mother. They surround the blood vessels, even when the blood vessel here has penetration into the brain, the pial cells follow it down, but not when it becomes a capillary. They disappear. But that blood vessel never contacts the brain cell, because of these glial cells.
This is a picture of an astrocyte, and you see the astrocytic endfeet, abutting the pia here, so we call that the pial-glial membrane at the surface. It's not pial cells contacting the brain cells, the dendrites. It's contacting the endfeet of glial cells and the astrocytes. And similarly, the astrocytes abut the blood vessels. So the astrocytes are critical in the oxygenation of the brain and the nutrition of the brain. Access to glucose and oxygen has to involve these astrocytes.
Now, there are other glia. I point out here, there are oligodendrocytes. We talked about how the oligodendrocytes make myelin. They also forms these little satellites around neurons.
And for any of you that want to study it, you can take a magnifying glass and study the picture. You can actually make out all those things here. You can see pial cells, collagen fibers, smooth muscle-- very thin. And note here, you have a blood vessel. And there's the nucleus, the blood vessel. There's smooth muscle.
And I want to quickly get on with this. I want to go-- it looks like I didn't open. Yeah, OK. This is all we're left with.
All right. So now we can get to the hindbrain. We have enough time, I think, to introduce the hindbrain and talk about segmentation of the hindbrain. And I particularly want you to learn about the differences between hindbrain and spinal cord. I call the hindbrain a glamorized spinal cord-- very similar in organization to the spinal cord, but you have to learn what the similarities are and what the differences are.
So these, remember, are the names for the encephalon, which is the Greek word for brain. Hindbrain, the rhombencephalon, the mesencephalon, the prosencephalon, consisting of the diencephalon and the telencephalon.
OK. So, first of all, when that neural tube forms at the brain level, it does something that is not true down in the core. It develops flexures. Now, what are the flexures of the neural tube? These are from the Nauta book.
Very early, you get these sharp bends. At the cervical level, this is the cervical flexure here. And there's the mesencephalic flexure. So they're both concave downward. Convexity is at the dorsal surface. And then in between those two flexures, you get the pontine flexure forming, and that forms in the opposite direction. And it's when that pontine flexure forms that the roof plate widens. It's like taking a pea pod that splits when you bend it. So it widens out.
Now, we know about the origin of the term rhombencephalon because of this rounded shape. We've mentioned that before. But there's another question I want to ask about that, because this landmark, the obex, is commonly used in neuroanatomy. The radiologists use it too. If you're in medical school, you're learning a little bit about radiology of the brain. You'll learn this.
This is what they mean by the obex-- this point right here at the caudal end of that rhombic shape, where the widened roof plate is no longer widened. That is what the obex is. It's an important area because it's an area where there's a weakening of the blood brain barrier. And it's very important for getting rid of toxins, it can elicit vomiting and so forth, when there's something bad in the blood.
OK. So these are now some topics that we'll be going through. We won't get to all of them today. We're going to compare the structural organization to spinal cord, talk about basic functions and cell groupings.
So first of all, how is the hindbrain embryologically very similar to the spinal cord? We want to compare and contrast the columns of secondary sensory cells and motor neurons of the hindbrain and spinal cord.
If I asked you in just a very few words what is the difference between hindbrain and spinal cord, what would you say? I mean, what are the secondary sensory neurons of the hindbrain? What are hindbrain senses, like auditory, vestibular? They form clumps of cells, nuclei. That's not true in the spinal cord.
In the spinal cell, there's a continuous column of secondary sensory cells, but that's probably because the inputs don't come in through a single nerve. They come in through multiple nerves. The dorsal roots of those nerves have a distribution that-- it always overlaps with the adjoining root. So there's a continuous group of secondary sensory neurons.
So let's look at that glamorized spinal cord. There's differences in the roots too. I mentioned this before. We have the spinal roots with a [INAUDIBLE] of roots, sensory in the back, dorsally. Motor in the front, ventrally.
But in the hindbrain, you have mixed nerves also. There are nerves just like dorsal roots. There are nerves like ventral roots. But there's also mixed nerves, where you have sensory and motor.
So in the book, I used colors for this, separating secondary sensory nuclei at their cell groups in the inner plate and similarly for the motor neurons. In the spinal cord, they're pretty continuous, unless we're dealing with the enlargements where there's extra goops of motor neurons innervating the muscles of the arms or the legs and the feet and hands.
So let's look at that. The picture of the embryonic spinal cord at the top-- embryonic hindbrain at the bottom, where the neurotube is thicker. You have the widening of the roof plate. But there, the secondary sensory cells form a column. They're always in that region, whereas here, they're in these separate columns.
Except when I picture them, I generally show different colors. I show, for example-- I'll then use red for this, because it's viscerosensory. And I'll use green here for the special century, and usually another color, green, for general sensory. Often the somatic senses are called general sensory because they cover the whole body surface quite generally, where as the special senses are more localized in the head region.
And then similarly for the motor neurons. We have the visceral motor neurons here. And then we have somatic motor neurons, like the motor neurons innervating the eye muscles, or the tongue. They're in that position. And then we have the branchial motor column here that are very much like the somatic motor column, but you can see, they form a separate column of cells and they have a different type of embryonic origin.
So let's go to functions a little bit. Hindbrain is known to be an essential controller of vital functions. And what vital functions are we talking about? Why is it that if you suffer much of a lesion or a stroke at the hindbrain, you're likely to die? What are the vital functions being affected? What is the most vital?
Probably not heart or blood pressure control, even though that's important in the hindbrain. But remember, the heart has its own ganglion, and it has spinal innervation. And even without that, the heart will keep beating.
Breathing, exactly. You can't breathe without circuits in the hindbrain. Those circuits even reach the midbrain. So all the way down from lower midbrain all the way down to the fourth cervical level, critical for breathing control.
OK. What are the other routine maintenance functions of the hindbrain? There's actually a lot of them. But what are some of the things that-- remember, what we called routine maintenance functions before? They're always reflex-type functions, or at least automatic. They can be programmed movements too. Yes? You have to speak loudly, or I can't--
AUDIENCE: [INAUDIBLE].
PROFESSOR: Yeah. Yeah, exactly. The vestibular reflexes. We don't think about them. They're pretty automatic. They keep us upright. And they're happening all the time. They're part of that mantle we're always wearing, the mantle of relexing. That's a routine maintenance function.
But there's other things too, things that bother us, something in the air that makes you sneeze. You just sneeze. It's a reflex, and you have trouble controlling it. Although, yes, we have descending connections from the forebrain and we can try to suppress it, but it's almost impossible to completely suppress.
What's another one like that? Eye blink. The eyes blink all the time. You can voluntarily blink the eyes and you start thinking about it. You won't be paying attention for a while. But it's a fixed action pattern. It's being generated all the time. It's very important for lubricating the cornea. Yes?
AUDIENCE: [INAUDIBLE].
PROFESSOR: Oh, there are. It's also a reflex. You can trigger it. But what you're really doing is you're tremendously increasing the input into the control of that fixed action pattern. You've greatly increased the likelihood that you will blink. But yes, it does respond to sensory input. In a very powerful way, that's true of almost every drive, every motivation.
We have a very strong motivation to blink our eyes. That's what a staring contest is all about. Can you resist? How long can you resist? Can you resist longer than your friend?
OK, well, how is the hindbrain involved in higher functions like speech? Well, wait a minute. Hindbrain is lower level, right? Well, it's involved in speech. Why? What do we need in order to speak? Respiration, important. Control of tongue and jaws and lips, all controlled by the hindbrain. Several different cranial nerves, as you will see.
What's the critical role of the hindbrain in feeding behavior? We can't eat with this hindbrain. Swallowing. It's a very complex reflex. It's a fixed action pattern. We call it a reflex, but it's actually a fixed action pattern that can be-- the probability of triggering it goes way up with certain stimuli, the stimuli in the back of the tongue. But we do swallow automatically and periodically all the time to keep our throats lubricated.
But what else do we need in the hindbrain for feeding? Control of the jaw-- jaw opening, jaw closing. There's a special nucleus that's part of the fifth nerve that's not just sensory. It's not just the trigeminal nerve. It's also the masticatory nerve, the motor complement of the fifth cranial nerve. Yes?
AUDIENCE: [INAUDIBLE].
PROFESSOR: That's always an interesting question, because a lot of it seems to be-- we do it pretty automatically. But you can become quite conscious of it also, just like for eye-blink or jaw opening. We'll do it. Even an unconscious person will show some of these movements.
AUDIENCE: [INAUDIBLE].
PROFESSOR: When you're doing other things, yes. But some other things can inhibit that. And that's even true of things in the gut, if it has an enteric nervous system controlling them. But some of them can be inhibited. But mostly that's automatic too. It's when the sympathetic nervous system is highly activated. It inhibits their system. Yes?
AUDIENCE: [INAUDIBLE].
PROFESSOR: Visual saccades. Yeah, that's a very good question. It's called a visual reflex because they are being generated all the time, but strongly influenced by the attentional mechanisms of our neocortex.
All right. So this just summarized most of what I just said. I didn't mention here the widespread modulation of brain activity in sleep and waking, arousal effects. But let's just see that a little bit from the neuroanatomical side.
First of all, neuron types-- Nauta and his collaborator, Ramon-Moliner made a nice contribution to this field when they wrote an article called "The Isodendritic Core of the Brain Stem." They were talking about two types of neural shapes that you keep seeing throughout the brain stem, especially in the hindbrain.
What are the two types they're talking about? What they called isodendritic is something that looks like a motor neuron-- long dendrites. There's the nucleus, there's an axon, and here are the dendritic branches. This is isodendritic, very much like a motor neuron. I won't try to draw the whole thing.
A lot of the reticular formation cells are like that. They just look like motor neurons. Though some of them are-- the dendrites are mostly in a plane. That's isodendritic.
Then they talked about idiodendritic. Let's draw an idiodendritic cell. Here is the nucleus. And here's a dendrite that instead of this motor neuron-like stellate shape, it's much more specific. And idiodendritic cells look very different. For example, it may be like this. It may terminate in specific layers. There's many idiodendritic cells in the retina like that. They did get different kinds of input in different layers.
Other idiodendritic cells have other, but not necessarily laminar, in the way they terminate. Cortical pyramidal cells we can call idiodendritic. They're specialized. Some interneurons, they're given names according to these very particular shapes they have. They're not isodendritic.
In addition, the Scheidels, who were great anatomists using the Golgi method here in America-- much of their career was spent in Los Angeles-- they described in addition segments in the neuropil. And they described axons with very wide distribution.
So here is a little diagram of these little disc-like segments. Here's what they found. Here are the isodendritic neurons that are renticular formation, just a few of them. But note, their dendrites, they found, are always within or mostly within these little plates.
And the axons from the cortex-- here's a few pyramidal tract axons-- note that they leave at right angles, and they go up into those segments, so you can diagram it. If this is pyramidal tract down here, the axons go up and terminate within those little segments. And that's what you're seeing in that picture.
Note here that the cells in the hypoglossal nucleus, the 12th cranial nerve nucleus, they're not in these segments at all. So they look very different in the Golgi state.
Now, here's the other thing they described that was so important. Here is one neuron. This entire picture is of one neuron. It goes from the cervical spinal cord to the rostral end of the thalamus, the diencephalon. And this is one thick section where they could follow the axon from this cell in the hindbrain-- it was a lower pontine cell or upper medulla oblongata. Here's the axon, and it bifurcates. Here's the descending branch where it distributes various collaterals.
And look at the ascending branch. Collaterals in the particular formation of the hindbrain, the midbrain where it has some extensive arbors in the central gray matter of the midbrain. And then it goes into the older parts of the thalamus. Widespread distribution, there's even a branch that goes into the hypothalamus. So with the number of neurons in the hindbrain like that, you can be sure that the activity of some of these cells is affecting the whole state of the hindbrain.
Now, a little bit about segmentation, how these segments arrive. you might want to know, where does that term come from, the branchial motor column? Well, in the embryo, there are what we call branchial arches. Branchial arches in aquatic vertebrates can form the gill arches.
But we still have that in our embryonic development. Here are the embryonic branchial arches. And each arch is innervated by a certain segment of the hindbrain. They're innervated by cranial nerve five, seven, nine, and 10, innervate these branchial arch tissues. The nucleus ambiguous is the nucleus that controls swallowing and vocalization. It goes out through the ninth and 10th nerve.
AUDIENCE: [INAUDIBLE].
PROFESSOR: Yes, the branchial arches are present throughout the vertebrates. But they develop later in the embryo into different things. So the branchial arches in humans here form the jaws. The auditory ossicles, the bones, the hyoid bone, and the pharyngeal skeleton, including the thyroid cartilage. These are all branchial arch tissues in humans.
So we know that the mesoderm below the head also segments, especially for muscles. These are the somites. Muscles form this segmented appearance. And early in development of the embryo, you can see them.
And we know now that these segments have different gene expression. And these gene expression studies have been carried forward into the brain where you keep seeing segments. This is one major type of gene, the homeobox gene, named for the sequence of genes in there, the sequence that these genes show that's similar, one to the other, but then they have differences too, so they're different homeobox genes.
They are transcription factors that are expressed differently in different segmental levels. And these are just examples. They've been highly studied in drosophila. And here they've colored the fly and shown the expression of different homeobox genes in different segments of the body.
And if they look at the chromosome that has those genes, they see that the genes expressed rostrally are at one end of the chromosome, the ones expressed caudally are at the other end of the chromosome, and so forth in between. The same is true of all the vertebrates.
But when you get to an animal like the mouse, you have at least four different chromosomes that have what we call paralogous groups-- that is, they're related to the homeobox genes in the fruit fly, but they're found in more than one chromosome. But still, if they're expressed rostrally in the body, they're at one end of the chromosome. If they're expressed caudally, they're at the other end of the chromosome.
This is just examples and actual photographs of the mouse embryos showing [INAUDIBLE]. Actually, they're immunostaining using antibodies that are specific for the protein products of the Hox genes that they're showing. So this is Hox B1. You see it's expressed in one little region in the brain, and then more caudally it's also expressed. Here, it starts its expression more caudally than this one. This is B4. This one even more caudally.
And again, there they are. There's their positions on the chromosome. And this just shows expression along the anterior posterior axis, how the rostral limit is very clearly defined. We'll say a little bit more about Hox genes right at the beginning of the next class.