Flash and JavaScript are required for this feature.
Download the track from iTunes U or the Internet Archive.
Description: This lecture completes the discussion about the evolution of multicellular organisms and the initial steps to the CNS of advanced chordates.
Instructor: Gerard E. Schneider
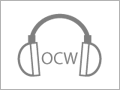
Lec 4: Steps to the CNS of ...
The following content is provided under a Creative Commons license. Your support will help MIT OpenCourseWare continue to offer high-quality educational resources for free. To make a donation or view additional materials from hundreds of MIT courses, visit MIT OpenCourseWare at ocw.mit.edu.
PROFESSOR: This is class four. I want to start with that simple neural tube we saw already in this little creature, amphioxis. The lancelets is another common name you can call that group of animals.
And ask this question-- we don't have fossils of the brains. Brain is soft tissue, doesn't leave fossils. So when most of our ancestors are extinct, how do we know anything about brain evolution? Somebody want to tell me? How much can we tell about the brain from the skull?
AUDIENCE: [INAUDIBLE].
PROFESSOR: That's right. Exactly. Another comment? OK, I can hardly hear you because of the background noise.
AUDIENCE: [INAUDIBLE].
PROFESSOR: And you can see evidence of that in skulls, that's right. So we know. But there's another factor that nobody's mentioned. Yes?
AUDIENCE: [INAUDIBLE].
PROFESSOR: Let's talk about development next time. We might get to it at the end of this class, but that's important. It's very important, and we will talk more about it. Did you have something else? No, you go ahead, since she's already--
AUDIENCE: [INAUDIBLE].
PROFESSOR: Exactly. And do you know how we judge how primitive they are? You can do a lot from skulls if you have the skulls, because they look the same as skulls. We can pretty much identify the species by skull details and skeletal details. And if they're relatively unchanged over millions of years, we can get an idea of how ancient they are.
And then, if we can get any DNA, we can actually just-- this is, of course, fairly recent. You can learn a lot about their age because the rate of mutations is fairly steady. And if we can get an idea of what their proteins were like from the DNA, we can learn a lot about evolution [INAUDIBLE].
But anyway, I have another question here. In the early evolution of the chordate neural tube, what was it that led to the changes at the rostral end? We saw amphioxis, there's no real enlargement here. Turns out there are genetic differences, there are differences in details of neural structure. But what was it that led to a real brain? What was the basic thing about these bilaterally symmetric animals?
AUDIENCE: [INAUDIBLE].
PROFESSOR: Exactly. So yes, and that led to the evolution of specialized receptor systems, which then records special motor control at the rostral end. So both the sensory analyzing mechanisms, the inputs came in through cranial nerves now, which turned out to be a little different from the spinal nerves. We'll be talking about both kinds of nerves quite a bit in the class.
And then, of course, the motor apparatus they needed. But also, of course, they needed to maintain the stability of the internal [INAUDIBLE]. And that also got very evolved, even starting with amphioxis. There were secretory systems in that rostral end of the tube-- the predecessor to the pituitary and hypothalamus.
And the sensory inputs, the ones we think of the most, are the ones that come in directly to the forebrain, the olfactory and visual inputs. And then I've already mentioned the visceral control that also involved that area.
So here's my little sketch. It's a very simple neural tube. And then expansions at the rostral end. I just sketched here olfactory and visual inputs. I think the figures in the book were a little bit better than this, but they were all based on these sketches.
And then I have a couple of the hindbrain inputs. Most of the cranial nerves come in through the hindbrain. There are cranial nerves for the midbrain too, but they're motor output. The third and fourth nerves come out of the midbrain.
But the midbrain became important in other ways. First of all, it got visual input, as we will see. Probably not the original visual inputs came there, but it also was a link between those forebrain structures, like olfaction, and the motor system, which was controlled by the lower brain stem and the spinal cord.
All right. So let's talk about now the expansions that occurred with these specializations at the rostral end. So brain starts to evolve. The hindbrain expansions in the chordates resulted from the evolution of adaptive sensory and motor functions. What's an example of a hindbrain sense? Input that comes in through a cranial nerve into the hindbrain, important sensory modalities.
What did we just show? What do those mean? So now the sensory will be talking about the trigeminal nerve [INAUDIBLE], the head region, especially the face. And then I have vestibular. I put vestibular in not auditory because vestibular is even more ancient. At least we think it is-- a lot of these things, there's a little bit of debate, and it depends on the details they find in the skulls. And it's been very difficult to get the very early period pinned down.
And I've asked you-- there's a homework thing relevant to that, and you'll be looking on the internet for some of that data. So here now, I've taken that brain with the three brain vesicles, and I've showed and expanded hindbrain. And we should keep in mind that, of course, along with these specialized inputs coming in, the brain evolved action patterns, movement control, that was new too. When it's a genetically controlled, a genetic controlled development of a circuit controlling movement, we call it a fixed action pattern, or an instinctive movement pattern. That's a term from the ethologists, but we can apply it to our discussion of the brain.
So let's now compare the specializations for case senses of a couple of fish, where this-- I like the illustrations from Herrick we're going to show. These are the Herrick brothers. Clarence Herrick and C. Judson Herrick were brothers, and they certainly helped establish the field of comparative neurology in America. That doesn't mean there wasn't similar kind of work being done in Europe and possibly elsewhere in the world, but they had these beautiful illustrations of fish.
What is the sense? Do you remember? Did you read it? What's the sense that led to some of the marked expansions of the hindbrain? Not trigeminal, not vestibular, but something else. Taste.
So these are to summarize the animals we're going to look at. I got the pictures from C. Judson Herrick's book, but they're actually all drawn originally by his brother. This one I take for comparison of the others. If you just look at this brain, the brain goes from this lower arrow all the way there to the olfactory bulbs, which are peduncle connecting the olfactory-- it looks like eyes, but they're not. This is all central nervous system.
And the midbrain is actually the widest part. And behind it, between the arrows, is the hindbrain. Now, keep that in mind and look at these other fishes. This is the moon eye. Look at the buffalo fish. You look at this, and you're used to seeing pictures of human brain, and you see something like this, and what on earth are we looking at?
Well, we're looking at changes in the neural tube, expansions for specialized sensory motor control. We call this in the buffalo fish, the vagal lobe because the input to it comes through the vagus nerve, the 10th cranial nerve.
Now, the 10th cranial nerve in vertebrates generally carries sensory input from the viscera, but the visceral sensory neurons get that input. The rostral part gets taste input, coming from the throat, the palate and the sides of the throat.
In humans, there's a little bit of taste input still coming. This animal has a specialized palatal organ. They filter-- they draw in little particles of the debris, the mud, at the bottom of lakes and rivers where they live, and they filter it. And they're basically tasting it. And there are specialized motor functions of that filtering apparatus as well, so they can select out the edible things in the water.
And they don't need a whole lot of vision for that. They just lie near the bottom and do their feeding. OK, now here's another animal that you're probably more familiar with-- catfish. This would be like the bull-heads many children catch when they go fishing when they're kids, but there are many other catfishes.
Again, strange-looking brain. But now the endbrains are a little bit bigger. They don't show the olfactory bulbs here, just the stalks that connect to them. And then you see the midbrain is still pretty wide. You didn't even see them. The midbrain here is not as distinct as it is in the mooneye. There's the big endbrain with the two tectal regions. There's the mooneye. Here's the catfish.
The midbrain is bulging out quite a bit. But now all of this is cerebellum. So it's more specialized in the cerebellum. But I want you to look at these bulges behind, because they all get taste input. There's the vagal low, much smaller than in the buffalo fish. Here it is in the buffalo fish. Here it is in the catfish.
But this bulge is called the facial lobe because that comes in through the seventh cranial nerve, and in this animal, much of it is carrying taste input. So let's look at their specialized taste system. There's a catfish. And this picture is illustrating just the seventh cranial nerve. It's a cranial nerve, and it is getting input from the head region, including input from these little marbles here that drags along the bottom as he's swimming. It's a taste organ.
But what is all this? It's the seventh cranial nerve distributing over the body surface of most of this animal. He tastes with his body surface, everywhere on his body. And it's all innervated by a cranial nerve. So that seventh nerve is no longer just-- it's coming into the head region, into the hindbrain. But it's carrying taste input from all over the body. So again, this animal is not depending mainly on its eyes but feeding, but on its taste organs. And it's led to these enlargements.
Now, that point, the specialized sensory functions, require neural processing power. And that entails enlargement of the neural apparatus that accomplishes it. So structures get bigger.
So now let's go up to the forebrain and talk about what I call the first expansion of the forebrain in evolution. What caused the forebrain to start to enlarge? The olfactory system, we think, has certainly led to its expansion. There's other things happening in the forebrain, mentioning our secretory cells, the caudal end is also getting the visual inputs.
But the first real expansion of the parts of the forebrain we call the endbrain, the forward part of the forebrain, is because of olfaction. And I just note here that when you see drawings, I'm often going to-- like, here I show pathways on one side here and another side here. Keep in mind that almost always, these pathways are on both sides. But if I draw them on both sides, the diagrams can get very complicated. So I often show them only on one side. But you have to keep that in mind throughout the class. We'll often show them only on one side. It makes it a lot clearer.
So here you'll see olfactory bulbs and some of the connections of the olfactory bulb. They're coming in to more caudal parts of the forebrain. In this case, it's parts of the endbrain. They're not going clear back into that predecessor of hypothalamus. They're coming to a part that became the corpus striatum. In fact, it's the most primitive part of the corpus striatum. That area, the striatal areas, have outputs that control locomotion. How did it do that?
Well, olfactory sense isn't doing the animal any good if it can't control his movements, and it controlled movements mainly by connections from the striatum that went from there into the midbrain. There were no connections to the spinal cord, no connections to the hindbrain. They were shorter connections, which is generally true in these primitive brains-- shorter connections.
And here's an example of a striatal cell connecting to the midbrain, the caudal part of the midbrain. And there are neurons there that we know drive locomotive behavior. It's important even in humans. And then that area has outputs down to the hindbrain and even, in many animals, directly to the spinal cord.
There was something special about that connection in the striatum that led to a property of the forebrain that is absolutely critical to its evolution. And that is this-- that the striatal connections were plastic. They could be strengthened or weakened, depending on the experience of the animal. So here's that animal foraging. He's smelling things, tasting things. He's getting feedback about the area he's swimming through.
So he learns whether they're good or bad. He learns whether that's something worth approaching, or if it's dangerous. Sorry?
AUDIENCE: [INAUDIBLE].
PROFESSOR: That's another issue about neurogenesis and its role in learning. We have areas important in learning. It's true that two major structures, the learning centers on them both have neurogenesis-- hippocampal formation and the olfactory bulbs.
But in fact, the changes I'm talking about here are not an area of a lot of neurogenesis in the adult, the striatal areas. What you're talking about is happening in the tiny neurons right in the bulb. So don't connect learning necessarily with neurogenesis. But when I say they're plastic, there probably does entail actual morphological changes. Most forms of learning do.
This was the predecessor to habit formation in the higher vertebrates, including us. The corpus striatum, which Ann Graybiel's been studying for many years, it's a very important site for habit formation. It's the reason that area has, like cortical areas, expanded in evolution.
OK, let's go back to the midbrain now. I said, what structure in the midbrain has become greatly enlarged in most predatory TAS fish? And then I want to talk about the two major outputs of that structure. One descends and crosses the midline. The other has an uncrossed projection. So we've got another problem to deal with. Why do things cross at all?
OK. What is the structure I'm thinking of? What's the big structure in predatory fish? When you look at the brain, it's really obvious. There it is. It's a visual sense. It's the surface gets visual input. It's the optic tectum. And there you see it. And this is a barracuda, an animal you've probably heard of because sports fishermen like to fish for the barracuda.
But just look-- here's the olfactory bulbs-- not very visible. And when they remove the skull, they left some tissue around the olfactory bulb there. But there's the endbrain, which includes the corpus striatum and pallial structures. Because remember, it's still part of the neural tube. So it's a tube where the walls thicken, and there's fluid in the middle.
And the part above that ventricle we call the pallium, or the cortex. And the part below, which often develops quite differently, is where the striatum develops in the endbrain. But there's the tectum.
Here's a diagram that shows that its connection-- a connection that dominated in these predatory fish we're talking about-- a connection from the eye. And all of it comes from the opposite eye in lateral eyed animals, animals with eyes that sit out here. Even in the hamster, whose eyes look about 60 degrees out-- so there's some overlap in the visual field of the two eyes. They still have mostly a cross-projection like this. And it goes to the midbrain tectum.
It allows an animal-- vision was so important, it led to marked changes in the brain. It was one of the two forebrain senses, olfaction and then vision-- because it gave something even better than olfaction did. Olfaction couldn't respond to things at a distance, but look at vision. It could get advanced warning. It could escape from things. It wasn't just predators that this was important for.
And just another picture of it here. I point out that two major outputs that are so important in evolution, of that tectum of the midbrain. One of them controlled anti-predator behavior, turning away. And the same pathway not only controlled turning away, but it went to the locomotor system that I mentioned before-- that same region that was getting input indirectly through the striatum from the olfactory sense and triggered rapid locomotion.
So now you have the means for the animal to respond by turning away and running, not just running, which could lead indirectly into the mouth of the predator. So he turns away and runs.
But there was another pathway that developed as this projection gained a topographic organization, they developed the ability to turn towards things-- very important for finding food. And of course, for prey animals, for predators rather, it became particularly important. It's the only way they could catch prey, is if they had a highly evolved system through rapid orienting towards the prey. And this is the main structure for that early in evolution.
So then here, this question-- why do the pathways from each eye to the midbrain cross to the opposite side like this? Why does that happen? I've never read a neuroanatomy book that actually has an explanation.
But I have an explanation because I think it came from my study of sociobiology and animal behavior. I paid a lot of attention to evolution. The reason there's no explanation, even suggestions about why crossed pathways developed, it's because people weren't thinking in a Darwinian sense. They weren't thinking of Darwinian evolution.
Anatomy developed within the field of medicine, and they were concerned with humans and comparisons with humans. So they weren't primarily thinking of Darwinian evolution. OK, I will just give you a very simple answer to this. We're going to go through it in more detail when we talk about the hindbrain, because it involves the somatosensory system as well.
And this is just a little summary of the hypothesis, but to cut things short, they must have developed across pathway because it was more adaptive. It had to survive and reproduce in some way. So my proposal is, it allowed more rapid escape behavior. So without a synapse, they crossed-- contacted descending pathway that controls escape behavior. And that's the proposal that I would develop when we get to the hindbrain, when we will study how-- because it's not just the visual pathways that cross. It's the somatosensory pathways from our entire body are crossed.
That's why everything controlling my right hand here is on the left side of my endbrain. I didn't say all of the brain. I said the endbrain, but also the midbrain. The midbrain and forebrain we have cross projections. Hindbrain and spinal cord, we don't.
So now let's talk a little more about that expansion of the forebrain-- less likelihood have led to a second major expansion. It's already started to expand because of the importance of olfaction. Olfaction was so important in feeding and mating behavior, in knowing whether an animal was a male or a female, whether the female is receptive for mating. And so important in finding good food and discriminating it from bad food and doing so efficiently, not having to wait until you taste it. Yes? Speak up.
AUDIENCE: [INAUDIBLE].
PROFESSOR: Sometimes they are. But in most cases, it's when certain genes will disappear from the population of genes. Those genes will disappear, and others will be preserved if there's any slight advantage. And for something like escape behavior, it only has to be small fractions of a second to make the difference between being caught and getting away.
And it's because of that-- if you just watch the films on WGBH on escape behavior and predatory action and look at the high speed films they need, and you realize how split-second timing makes all the difference in survival. And that's what I'm basing this kind of thinking on. But we will bring this up again, and we'll talk a little more about it. I just want to give you an overview now of these brain expansions.
The second major expansion-- another equally important. We had to have a forebrain already, and we know it must have been olfaction that led to its expansion. But we know now the forebrain is [INAUDIBLE] more than the olfactory system.
Other senses came into the forebrain. I already mentioned the big advantage now to the olfactory connections to the striatum, they were plastic. The connections of the visual system to the midbrain, as big as it was, were they plastic? Not in the same sense. Their short-term plasticity, habituation, sensitization, it doesn't last very long. But the kind of learning allowed habits to form, long-lasting habits.
The other senses, I think, in evolution started coming into, invading the endbrain for that to take advantage of that system. They come directly to the striatum. There are visual path-- and most of them come this way. Here's a link in the midbrain. It's growing to neurons in what we call the tweenbrain. Most of them don't go directly from midbrain. Almost always, they'll go through the link there.
And these then go into the endbrain-- the striatum but also the pallium. Let's just talk about the striatal ones, because that's the ones we've talked about already. There's great advantages to be able to learn and remember habits based on visual input and auditory and taste input-- so input coming from more caudal structures.
Now, the visual input, some of that came directly into the tweenbrain. The eyes actually involved from the tweenbrain. So this pathway I'm showing from the midbrain certainly wasn't the only one carrying visual information into the striatum. Some visual input went right through a part of the diencephelon. We called it the subthalamus, which projects directly to the striatum, as does the older parts of the thalamus.
All right. So I think that's what led to the second expansion. Then I say a third major expansion of the forebrain has occurred in mammals, apparently because of the evolution of another structure. You should all know what that is. What is the huge part of the human brain? The neocortex.
And what in a thumbnail here does it do? I'm mentioning here the various functions that led to these changes in the neural tube. But the one that involves neocortex is this last one, systems for anticipating events and planning actions, the things we call the cognitive systems.
I'm not going to mention communication, language, and all of that. That was a very specialized things that happened in humans. This is mammals in general. And I'm describing here those systems for anticipating things. We call on the sensory side, sensory images. And I use that very broadly. We have auditory images, visual images, some of the sensory images, and for planning and preparing for actions-- all non-reflex types of functions involving some kind of internal representation of the external role.
That's what the neocortex is specialized for. And I hear mention, something you'll hear a number of times in the class, that yes, some animals don't have very much like a neocortex, like the birds. But there are non-neocortex structures that accomplish similar functions, because they have connections like the neocortex. So we'll be encountering that and discussing that a little bit as the class goes on.
So this was a third major expansion. And it was the neocortex that expanded, especially-- I just mentioned here-- the part in the higher animals, especially the primates but other animals too that expanded the most, certainly in primates, was what we call the association cortex. And I develop the idea in the book that that association cortex that's expanded the most in more recent evolution is actually-- it has properties like the earliest cortex, the earliest pallium, not the most specialized cortex that evolved in between.
OK. But you'll see my reason for that as we go on. And of course, as the cortex expanded, the cortex projects to everything-- projects heavily to the corpus striatum that led to the expansion of the striatum that projects to the cerebellum, and led to expansions of the cerebellum.
So here I just depict that. I take this primitive animal with a somewhat expanded forebrain, a pretty big midbrain. And I show how that endbrain grew. It grew a lot. And this would be like a rodent that we study in the lab.
And along with that, the cerebellum expanded. And it's a correlation that's pretty strong. The bigger that forebrain gets, the bigger the neocortex gets, the bigger the cerebellum gets.
And I had mentioned other functions here that I've not talked much about, controlling fine movements, especially with the evolution of distal appendages, the hands, the feet-- especially the hands can manipulate. That led to an evolution of one of these somatosensory areas that we started calling motor cortex as well as the cerebellar hemispheres. But the cerebellum gets input from all different areas of cortex, including the association areas.
So let's just talk-- we talked about expansions. Let's just talk a little bit about methods for comparing brain size in the various major groupings of chordates and talk about a major result of such comparisons. What is the method I'm thinking of? Yes.
AUDIENCE: [INAUDIBLE].
PROFESSOR: Yes. We almost always will take the ratio. We talk about relative brain size, because we're comparing it to body weight. That's because the size of the body is such a major determinant of the size of the brain.
Now, there are exceptions for individual structures, but in general, as body size expands, so does the brain. And you plot brainwave versus body weight, and it usually is done on log-log scales, like here. Not showing individual points. Here's a point for mammals. You can see they plotted here on the ordinate, the size of the brain, and they plot the body size here. Here's the previous one. Here's the body weight in grams in logarithmic scale. Here's the brain weight in grams. And of course you can take volume just as well.
And here, the different colors show the envelope of points for many different species of mammal. They're all in that green area. And here, the purple area down below, it's lower in general. But it still is along this diagonal line. That's the cartilaginous fishes, the sharks and the whales.
And here, the upper part that's getting close to mammals, but a little bit below. It's the birds. And then this is a very large group, the ray-finned fishes that some of those are-- it's almost reached the birds here. And even just a little bit of overlap with some mammals. And then reptiles. And the lowest here are the jaw-less vertebrates. But again, a lot of overlap with that huge group of ray-finned fishes.
And this is just for mammals-- and this one-- just a few points done to emphasize in blue, they're showing individual primates. And they've selected a few other animals, like porpoise because it has, in relative terms, a very large brain with respect to its body weight. But if I tell you that humans have relatively the largest brain, it's because the point is the farthest from that line. So relative size diminishes as we go in this direction and increases when we go in that direction. That's how you read those graphs.
So you can see here, the animal with the largest brain of all living creatures, the blue whale. There he is. There's the elephant, also larger by far than human. But in relative terms, not as large. So that's the method.
I would like to-- in a few minutes, because I've got a lot of slides for the next class-- I want to start discussing it now. We've already mentioned maybe a few things. You talked about development a minute ago and wanted to related it to giving us some evidence about brain expansion, brain change in evolution.
There's been a lot made of that issue. You'll hear the phrase that ontogeny recapitulates biologically. What does that mean? What do the terms mean? And what do we mean by a phylotypic stage of development?
This has been very popular in the earlier history of neuroscience. It's based on this kind of picture. This was drawn not by the original discoverer of the relationship which is by von Baer, a contemporary of Darwin. In fact, Darwin cites the idea in his book The Origin of Species. But I looked for it and found out he doesn't cite von Baer. He cites Louis Agassiz improperly.
But Ernst Haeckel took up that idea a little later and did studies and came up with these pictures. He's taking these species you see at the bottom-- human, rabbit, cat, hog, chick, tortoise, salamander, and fish-- all looking very different in many details, anyway, when they're born.
But look at them very early in development. This is after gastrulation. This is well after neurolation. And they're looking pretty similar. The only trouble was that Haeckel didn't look at every species. And sometimes he wasn't very accurate in the way he drew things.
So it's still a relationship. Haeckel took it to extremes. This is the way he shows it-- very different in the early gastrula stages. Then he said if they were a member of the same phylum, like the chordates, they all went through a stage where they look like this. And then they develop differently into different species after that. And these were the pictures he drew just to illustrate the data for that.
So even though there are really marked exceptions to it, the general relationship certainly leads you to believe that if you look at the nervous system in these early stages, they do look pretty similar in widely different chordates, even though they end up involving quite differently in different species.
All right. So it leads us to at least expect many similarities in the CNS of all the vertebrates anyway. And these have been found. I've mentioned cynodonts already. What is a cynodont? Why is it important in the story of brain evolution? Why do I bring it up? Cynodonts have been extinct for some time. What is a cynodont? They existed for a very long period. They precede most-- sorry?
AUDIENCE: [INAUDIBLE].
PROFESSOR: No, they're not dinosaurs. But they overlap with the dinosaurs. They did exist throughout the entire Jurassic period, but earlier. They existed through for geologic eras. Yes?
AUDIENCE: [INAUDIBLE].
PROFESSOR: Exactly. The mammal-like reptiles is the more general way we refer to them. These are the mammal-like reptiles. But yes, mammals appeared and evolved during the period of the dinosaurs as little creatures, running around the forest floor. It was to their great advantage to be nocturnal, because so many dinosaurs were diurnal and had very good vision. So to avoid predation by those big reptiles, they had to occupy a different environmental niche.
And it was among the cynodonts that we could say that the sketches I'm going to use were like an early cynodont, because the later cynodonts were really very similar in many ways to reptiles. But skull features show them still not to be mammals. And they go by a number of different features to specify how mammal-like they are.
OK, well, we'll come back to this next time. And we'll be talking about what I think is like a cynodont brain, but you could also call it, like, an amphibian brain. The sketch would fit either one.