Flash and JavaScript are required for this feature.
Download the track from iTunes U or the Internet Archive.
Description: This lecture is the last in the course and summarizes brain development and plasticity.
Instructor: Gerard E. Schneider
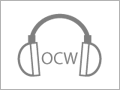
Lec 38: Plasticity in brain...
The following content is provided under a Creative Commons license. Your support will help MIT OpenCourseWare continue to offer high quality educational resources for free. To make a donation or view additional materials from hundreds of MIT courses, visit MIT OpenCourseWare at ocw.mit.edu.
PROFESSOR: Just a little bit from last time. I won't say too much about the Polyakoff work on cortex, but you'll notice that like Mesulam, he has three kinds of cortex, except his third kind, which he says is the most advanced area of cognitive functions, he includes a lot more in this area, in human-- would include a lot of Mesulam uni-modal visual association cortex. That's the major difference.
The other thing to note is just like most other people, they indicate that that most advanced cortex doesn't exist in rats and hedgehogs and similar animals. It's very little even in the dog. But remember what we said-- the area where our highest functions are localized, they are the multimodal association areas, which we postulated that multimodal cortex is the most primitive cortex. But it's the area that has expanded the most.
The brain becomes far more compartmentalized and specialized with evolution. Human brains are probably the most compartmentalized, most specialized of all the animals.
A little bit more about these that comes out of that Moscow work and some other topics. First of all, postnatal growth of different territories. He shows the amount of postnatal growth on the ordinate here, and he's just studied that, made measures for different regions of cortex.
Just note here the first four bars are all multimodal association areas. F means-- he says frontal areas, but he means the prefrontal cortex-- middle, temple, inferior parietal, and the temporal parietal occipital area that's an area in between this multimodal cortex and the area [INAUDIBLE].
The second thing, then, is myelin genesis. This is a picture from Cecil and Oscar Vogt, who studied that. The areas to get their myelin first start in the heavy dots and the lights are the areas to acquire myelin last. You see that the multimodal association areas tend to be the areas that have expanded the most postnatally. The areas where we've been localizing the highest functions in humans tend to myelinate late.
There are some exceptions in the limbic areas. This is from the work of Paul Flechsig. The numbers here in his pictures don't represent broadening areas. The numbers represent the order of appearance. Number one is the first place he saw myelin appearing. Number 45 is the last place. 45 here in the lateral prefrontal area in humans.
You see the early numbers in the primary visual area, primary sensory and motor areas. Primary auditory areas, they all myelinate very early, and you saw pictures of that way back when we were doing chapter six when I showed a picture from the work of Flechsig, a photograph of one of his sections of the human brain, a horizontal section.
This is just the growth of the thickness of the association layers-- layer three. Here's prenatal growth of these five different areas, and I pointed out what they are from y fields, prefrontal cortex, and the Broca's area. They're all in the frontal lobe.
Note here that these areas are still becoming thicker after age 15. He just has 15 and then the put the rest of them into the adult category. They're not completely finished growing even in the mid-teens. I said 15, but it's 12 years.
I just want to mention Larry Benowitz' studies of these areas, like layers two and three. That's where he found the protein that is often referred to as the growth-associated protein because it's present in axons during early development. Fallout zones have a lot of growth-associated proteins. It seems to be correlated with periods when processes have these filopodia extending. Growing axons always have those.
I studied it with Larry Benowitz in the hamster optic track, for example. We found that it's located all along the axon very early, and then when the arborization is occurring in the tectum and geniculate body. The gap 43 becomes localized to the terminal region and then declines after the maturation of the axons.
But even in adult humans, Larry finds that protein localized in these associational areas, and those are the very areas that have been found by [? Ellie, ?] and now other people are confirming that-- that throughout the cortex, they look with modern imaging techniques and image cells over an extended period of time. They see remodeling happening, a lot of changes.
We think that those many small neurons, many interneurons, mostly gabaeric interneurons. Whenever they've tried to localize the cell part, they find that there are these GABA-containing interneurons, the very neurons most of which don't originate in neocortex, in the neocortical ventricular layer-- they originate in other areas.
OK, we don't have time to talk about dominant and non-dominant hemispheres. I just want to point out that they are anatomically a little bit different. Sometimes, the differences are rather large, like in the area around the auditory cortex, areas related to understanding and speech and production of speech. That work was done right here in Boston when Norman Geschwind, the famous behavioral neurologist, was still alive and his associate, Al Galaburda did those studies, and he's still working at the Beth Israel Hospital.
The ideas on thalamic evolution, I'm just going to let you read. I've gone over them before. I just summarized them in these two slides.
I want to go on and talk about development and plasticity in the neocortex. One thing first, very relevant to this topic-- this just came out fairly recently in the February 20th issue of Cell-- neurogenesis in the striatum of the adult human brain.
Here's what they did. They used carbon-14-- natural labelling of humans exposed to carbon-14 due to atomic testing. They're eating foods and so forth. Some newly-generated neurons end up being labeled with carbon-14. If some sensitive measures define them, they can get measures of neurogenesis.
They can see that rostral migratory stream that we know from studies of rodents migrates into the olfactory bulbs and that's why you get turnover of the small granule cells, the most numerous cell type in the olfactory bulb. I think it's around 40-day period there. They could turnover completely. There's a lot of generation.
This study says that in humans, those cells get diverted into the striatum. In the rodent, they go right past the striatum, they're generated in the lateral ventricle-- the ventricular layer there around the lateral ventricle. But these don't continue into the olfactory bulb. It wasn't clear to me.
I haven't even completely read every detail of the article. It's not clear whether any of them get into the olfactory bulb in humans. That would be a big surprise if that were true, but it's a fascinating finding if it can be confirmed in other labs because it would indicate that in each of the two major forebrain systems for learning that we talked about in this class-- the hippocampus and the corpus striatum, and that includes for learning both dorsal and ventral striatum. He talks as if the new neurons are all going into dorsal striatum. I'm not sure that that will turn out to be true. The point is, new neurons are going right into the striatum in humans.
Of course, now we have to look carefully to see if there's none of them going to the striatum in other animals. I would suspect in other very large animals that they probably do, but so far, we only know about human because the studies just haven't been done yet.
AUDIENCE: [INAUDIBLE]?
PROFESSOR: Sorry?
AUDIENCE: [INAUDIBLE]?
PROFESSOR: The carbon-14 data dating was done they were alive, naturally, because they were exposed to-- the fallout contains carbon-14. A natural experiment. Unnatural, actually, but it's atomic tested, yes.
AUDIENCE: [INAUDIBLE]?
PROFESSOR: Very, very interesting question. Could be exposure to radioactivity itself caused some abnormalities. We don't think so because the levels are very low. But it's still an interesting question, and I hope it leads to a lot more work because we've only recently seen a lot of work on hippocampal turnover and its relationship to learning. We know that animals are learning a lot and, exposed to enriching environments, do generate more cells. We know the stress reduces cell turnover.
When we talked about development, we had these four major events-- neurolation and the formation of the neural tube. That is formation of the neural plate and then the neural tube. And then proliferation of cells, and then migration, and finally, the growth of axons and the dendrites that we call the differentiation of the cell, the adult cell.
These are questions here. First of all, what is the subpia granularly? When we talked about proliferation, we talked about cells dividing in the cell cycle. You see them synthesizing DNA here and then splitting into two, and they split in one of two ways, either symmetrically or asymmetrically, and that had different consequences because proteins are not distributed the same throughout the cell.
Proteins tend to be distributed differently and nearer the ventricle than away from the ventricle. If they split asymmetrically, they tend to have much more of that protein in just part of the cell. The ones that are further from the ventricle become post-mitotic. They migrate towards their adult locations and the others stay mitotic until the end of the period of proliferation.
OK, but what is the subpia granular there? You could say these are granule cells. They're the generating cells of the ventricle. They're very small cells. That's why we call them granular. The subpial granular layer, you may know, exists in the cerebellum in all the animals that have been studied.
Cells migrate from the rhombic lip area of the hindbrain, the other plate of the hindbrain, and migrate up into the cerebellar cortex. But then they keep dividing right under the pia and the cells that are generated in the pia then migrate down into the cerebellar cortex and form the granule cells for the cerebellar cortex.
Apparently, in larger-brained animals-- animals that have a lot more cortext-- again, there's really numerous small cells, granular cells, in the cortex. The larger the brain, the more of them there are. They develop this transient, generative layer below the pia in certain parts of the brain. That's the subpial granular layer. It's not present in the lab animals which are normally used. It's not present in the mice and the rats and hamsters.
Now, these two types of cell division were related by Rakic, Pashko Rakic. This just says what I talked about. This is one of Rakic's illustrations, but he pointed out that the period of symmetric and also of asymmetric cell division in the monkey is-- the periods are shorter than in humans. He said that even one additional symmetrical cell division should result in a considerably larger cortex. The human, just by continuing several more days, is going to end up with a much larger cortex. We know this is under genetic control.
What about asymmetric cell division? What should happen then? Well, what should happen is you look at his picture here. Here's the ventricle down here. Here it is in the cells chain. The cells are generated in ventricular and then also the subventricular layer.
The ones that are migrating due to asymmetric cell division as more continue to be generated by the stem cells down here, you can have many cells moving, as he shows here-- many cells moving along the same radial glial cell up into the cortical plate. The more asymmetric cell division there is, the thicker the cortex should become. The human cortex is considerably thicker than the monkey. We have a longer period of his asymmetric cell division.
This just illustrates these periods, and I summarize it here. He wrote about that back in '95, Trends in Science. I show pictures, one from Rakic, the monkey-- only the earlier periods where you see these different layers designated-- ventricular and subventricular zones where the mitoses are occurring, and then an intermediate zone, where there are migrating cells but they don't stay there. That becomes the layer of the white matter.
Then there's a subplate layer and a cortical plate. The cortical plate is where the cells collect. We normally call it the cortical plate in the period when you can't differentiate different layers. He shows some of them beginning to differentiate here and many of them not differentiating.
At the top, mostly process-- just a few neurons. Call that the marginal zone. And then he's got in parenthesis here the subpial granular layer-- that's what SG is. This is from our work, work I did with Janice Naegele in her Ph.D. work where we're showing postnatal day one, postnatal day five in an adult hamster and labeling those same things, except there's no obvious subventricular layer in the hamster. Animals with a larger cortex tend to develop that.
Notice here at postnatal day five, there's a layer five and six that have differentiated so you can identify them. But above that, we just call it the cortical plate because you can't see separate layers two, three, and four. Here's the adult. You can see all the layers.
We looked at that picture of migration. What do we mean by the inside out pattern of migration? It has to do with when cells undergo their final mitosis and migrate. That's the birthday of the cell. We don't say they're born yet when they're just generating. It's only when they become post-mitotic and they migrate. You say that's the birth date, when they finish mitosis.
The inside out pattern, this is the picture we were looking at. This is from the study at Harvard when Pashko Rakic was at Harvard. He had a very good technician that had come here from Vietnam, a Vietnamese couple that I helped in getting settled here, and then I found her a job with Pashko Rakic. She did this fantastic work with him where they labeled cells in the monkey by injecting the uterus of the mother at various stages. Here, you see this is e40, so this is about e45.
When they injected the tritiated thymidine at e45 and then waited until the animal's cortex differentiated, they found the labeled cells here at the bottom of layer six. If they waited much longer here to embryonic day 90, all of the cells that were labeled are up here in layers two and three. In between, you have this pattern-- earliest born cells end up at the bottom.
The later born cells then have to migrate past the others. They're generated from-- this is inside, near the ventricle. This is outside, near the pia. That's the inside out pattern. That's all we mean by it. The same thing has been discovered and was known earlier from the rodent work where they use other methods of labeling initially. It's also been done with tritiated thymidine for labeling the cells at their last birthday. There's other methods of labeling, too, that you can use.
What are the other proliferative zones other than the ventricular zone? We've mentioned them before. We talked about it mainly way back in chapter 12, where there's some illustrations-- two illustrations there towards the end of chapter. One of them is from Rakic, where he summarizes the primate compared with the rodent. The other picture is another summary from work on cells that aren't born in the ventricular layer in the rodent.
Remember where they come from? We had just been talking about Harvey Karten's hypothesis that the dorsal ventricular ridge cells migrate very differently in mammals and in birds-- a hypothesis that has been largely supported. But basically, the small gabaergic interneurons of the cortex come mainly from the striatal area, the area that is in the position of the striatum.
It's called ganglionic eminence in humans. In rodents, there's a very clear medial ganglionic eminence and lottal ganglionic eminence. Most of them come from the medial one, but they come from the lateral one, also.
For the posterior parts of the hemisphere, it's just one ganglionic eminence, so they call it the caudal ganglionic eminence. The ganglionic eminence region gives rise to most of these interneurons. These are the chapter 12 pages.
I want you to be a little bit familiar with the Finlay and Darlington publication of how structures change in size in different species largely as a function of the total brain size. In other words, the degree of concerted evolution, rather than mosaic evolution, is considerable.
I asked you how they actually do their plots-- just look at that. The question, really, is how much does the neocortex grow with regard to the whole brain in various mammalian species? You can ask, does the rest of the brain grow proportionally? Basically, it seems to-- in these plots on the ordinate, they take the logarithm of the size, the volume of a structure like neocortex or striatum.
Or the hippocampus down there, here's the medulla oblongata. There's the paleo cortex. There's the septal area. They plot it against a logarithm of the size of the entire brain.
Remember, these are logarithmic plots and they're taking, like for neocortex, the entire neocortex. They see the degree of concerted evolution-- as the brain grows in size, so do all these structures grow in size. Note that neocortex is growing more, and if you plotted on a linear scale and look at the left here.
This is still the log plot on the [INAUDIBLE] here on the ordinate in this 3D picture. You can see the huge amount of increase in size of the neocortext. That can sometimes be missed when you look at a picture like this. This is very, very large compared to this because of the logarithmic scale.
But notice also that the different colors are for different groups. Note here for the insectivores-- the size of neocortex, the relative size, falls below the curve for other groups. For insectivorous again, for the paleo cortex-- known as the olfactory cortex-- is actually larger in relative terms, compared to other animals. But still you have to say there's considerable concerted evolution.
But remember that when you deal with the neocortex-- the different parts of the neocortex-- this says nothing about it because there's tremendous variation in relative size of different parts of the cortext. This would say nothing at all about that.
The other part of their analysis was an interesting one. They did factor analysis to look at what correlates with these differences in size of these different structures? They found that two factors-- one represented by the blue bars, one represented by the orange bars, and you're looking at the amount of the variance explained.
That's what factor analysis does. This is the summary here. The blue bars here indicate that one factor accounts for over 96% of the total variance. It's highly correlated with neocortical size, least correlated with olfactory bulb size.
A second factor accounts for 3%, leaving only 1%. It's most highly correlated with the olfactory bulbs and next with limbic system structures, not correlated with neocortex size. There appears then to have been much more mosaic evolution in the olfactory and limbic system structures.
We already know how relatively huge the olfactory bulbs are in rodents compared with primates. That's just one example of this.
A little bit about nature and nurture in the formation cortex. We want to know how the cortex gets specified into its different areas. Before there was very much genetic work. There was a lot of arguing about whether there was genetic determination of the different areas.
It took a long time to get enough data because there were no possible explanations, just a pattern of axon connections could explain a lot. Actions come in the thalamus in an organized way and they distribute according to the way they're organized in the internal capsule. If their terminations then resulted in differentiation through the cortex-- differently, depending on what inputs they were getting-- that could account for quite a bit.
But the evidence for a genetically-determined map has been the most supported. But as we know, in every nature nurture issue, it's almost always moot. There's a lot of evidence for the effects of an experience of various sorts, as well. But basically, for more and more cortical areas, we do have specific genes that are active during our development.
The first one was work by [INAUDIBLE] that we discovered this limbic-associated membrane protein. Then there's been interesting studies of using transplants-- like if they took some limbic cortex that had this protein and they transplanted it to somatosensory cortext, the fate was it didn't become some other sensory cortex. It stayed limbic cortex. But the result depends on the age.
If you do it before embryonic day 12, before these proteins start to be expressed, before that gene is expressed and that protein is formed, then it just becomes somatosensory cortex. Similar work was done in the rat between visual area and somatosensory areas. You take a little bit of visual cortex and put it in somatosensory cortex. It becomes somatosensory cortex if you do it real early, but if you do it too late, it doesn't become somatosensory cortex. It keeps its specifity.
What about the epigenetic factors, like activity? The visual system's been used for a lot of this, and I want to know here about an example of how abnormal activity can affect the development of axon connections, even when the activity is prenatal. We think that vision must start after birth, right? What can an embryo in the womb see?
Well, it's making a big assumption that activity always depends on open eyes, and that, in fact, was found out to be true in Carla Shatz' lab. She gave a talk here just recently. She's continued to work on these issues. With her work with Meister, Wong, and Baylor, they discovered these waves of activity.
This is a little schematic of the retina that she's repeated, showing it at different times. She's plotted the time here. You see the activity beginning here, and then it moves right across the retina to the other side. This is happening well before birth.
She found that in fact, activity plays an important role in the formation of precise topographic and organized connections. Topography isn't determined by this activity. How precisely the axons are terminating is affected by it. That was the first discovery of this.
If pecks of activity had been worked on for some time in the lab where Carla was, Hubel's lab-- she was David Hubel's graduate student. The study of binocular connections. We've talked about the ocular dominance stripes in the cortex. These are just plots of relative response of single neurons to input from one eye versus the other eye. One eye in one side, the other eye in the other side, and then many neurons get input from both eyes.
You see how the neonate is different, and if you monocularly deprive the animal-- this is usually done with cats-- the the occluded eye, you end up with very few cells. When you open the eye and stimulate it, there are very few cells. Now, it can't respond at all to the occluded eye, whereas the open eye, most of the cells are responding. The connections must be changing.
This is basically a picture to summarize that where you see the diagram here just illustrating two layers, rather than all the layers, just to indicate the layers that respond to one eye versus the layers that respond to the other eye, and you see how early in development they just overlap in the cortex. They go to the right place topographically, but they just overlap with each other, right eye and left eye. They end up then segregating over time.
If you block activity, they don't segregate. You can completely block activity simply by blocking action potentials [INAUDIBLE]. They stay completely overlapped. If you get unequal activity in the two eyes, like patch one eye, then you end up with wider stripes for stimulated eye and narrower stripes for the other eye.
It happens in strabismic individuals. They can end up using one eye much more than the other eye, and the eye that they're not using very much will have much lower acuity. Acuity depends on the amount of cortex devoted to the sensory surface.
This shows you what's happening to the axons. These are in kittens. This is in layer four, so this is presumably a thalamocortical axon. It has all the characteristics of thalamocortical axon terminating in layer four, bottom of three. You see a very dense, widespread terminal arbor, but if you look in adults, this is one axon terminating in two different ocular dominance stripes. That's what happens with the activity of development in a normal adult cat.
Here's the effects of deprivation. This happens after just a few weeks. Here, you have the non-deprived eye, axons representing the non-deprived eye. Here's the deprived eye where the axons just don't have symmetry. That's long term.
Short term, and I think here, they got down to around week. Maybe it was up to 2 weeks. These are all up to months. I said weeks, but these were longer than that. These are the short-term ones, but you see they're getting the same effects, even after the shorter time. So it's happening pretty rapidly.
We think that the connections made by these axons are consolidated by the activity they generate and their ability to fire action potentials themselves. I think that Hebb's rule cells that are firing together. They're wired together. Seems to explain a lot of what's going on and we know there are many molecular studies of this in recent years.
There's other paradigms that have been used to study this. One is alter the wiring diagram by early brain lesions. I was able to do that pretty early using hamsters, but the hamsters are more difficult to do the physiological studies. So then it started being done on ferrets and other animals. The ferret was particularly good because it's born in a pretty immature state before the retinal projections are fully mature.
This was done by Mriganka Sur and his students. Here, he shows what happens if you just remove the inferior colliculus and superior colliculus. The axons are pruned and they're deprived-- the thalamus is deprived of its normal large auditory projection from the inferior colliculus.
What happens is axons of the optic tract that are passing over the medial geniculate will grow into the medial geniculate and terminate. That means you would expect a visually responsive area in the auditory cortex, and that's what they found by recording. They mapped it.
Here's the auditory area. The visual area would be way up here. The auditory cortex now has a topographic map of the visual field that's not quite as precise as normal, but it's there. And then when they looked at unit properties, the selective response to contours of different orientation. They found that that existed, too. Again, it was a little more sloppy, the tuning, but it could have been normal. But it was pretty good tuning.
We also know that the brain changes after blinding. This is from a study of the short-tail possum. This is the normal where you see the visual areas here, auditory areas and smell sensory areas getting mostly unimodal input with some multimodal areas here in between. Remember, they're saying the unimodal, and most likely, there's some multimodal input.
But look what happens if you blind them early. You get throughout the visual and areas around auditory and somatosensory. It's all multimodal. They were looking at the remaining somatosensory and auditory modalities and they were getting cells responding to both modalities in all of its cortex. Even in the primary auditory cortex, they were getting somatosensory units now. Within somatosensory cortex, they were getting some auditory responses, too. It's an amazing change.
There is other data on humans as well as on rats indicating that visual cortex takes on other functions after blinding. It's used by rats, for example, in solving mazes. They're using visual cortex to help them solve mazes. This kind of data indicates that that's not surprising considering what's happened.
A possible explanation is that normally, there are very precise connections, as I've argued before, that are multimodal. They're sparse, and they might be too sparse to actually drive the cells to trigger action potentials. But when they increase-- when the neural input is gone-- they could increase in strength. We call that the silent synapse hypothesis where once silence synapses suddenly become active, probably because they're sprouting-- they're increasing in their strength.
There are experiments now with mature monkeys that indicate something similar, just to help you make sure you're understanding that paradigm. First, look at the receptive fields in the hand. They're normally limited to one digit. We have good acuity in our fingers and monkey has the same. When you record from units in the hand area of the cortex, you normally get responses just on one digit.
But if you train them in a task where repeatedly, they have to grasp a bar and move the bar, they have to grasp it in a certain way like this. Multiple digits are touching the bar-- both the distal part of the hand and the more proximal part of the hand when they grasp the bar. They're trained-- not just for many trials a day, but over many days.
When they do that for a long time, then they get many cells with these multidigit receptive fields. Those kinds of cells weren't there before. Has there been rewiring? Has it been sprouting? This shows a map. I think this one I put in the book. The multidigit receptive fields in the distal part of fingers and the more proximal part of the fingers are represented by the red and the blue colors.
If you look at a normal, there's almost none of that. But in these trained animals, the cortex changes considerably in the nature of those receptive fields.
You could argue that it's quite possible that such connections did exist but then they're increasing a lot in strength, so now we can record their effects. Other people have thought that there might be anatomical changes, but it's been difficult to get any real evidence that that's happening.
OK, now these are just additional questions that you should be able to think about from what we've talked about and what you've read about. We've already mentioned today about changes going on in those superficial layers two and three. We think that the input to the hippocampus, it provides the hippocampus information about local environments, it remembers. The hippocampus forms long-term memories to be retained. They can't stay in the hippocampus.
We think most of that's happening in the parietal associational areas, the multimodal areas, and we think the changes are likely now, from the recent work, to be in those superficials areas. So far, there's not been studies of just how stable those changes are that they observe in layers two and three.
When you just look at them, they seem to be changing a lot, but when you get a long-term memory forming, we know these memories might change some and they may change a little bit when you're sleeping. In fact, you retain something very, very well over a very long term. We don't understand exactly how that's happening and why some things are more transient even in the neocortex and some are not.
I just talk a little bit about other areas of plasticity and especially here. We talked about it embedded in the corpus striatum, and that includes not just the ventral striatum, but the versal striatum. Both of them involve the dopamine projections. It happens in the cerebellum. It leaves shorter-term memories in the hippocampus. In the brain stem, even in the colliculus, you're going to [INAUDIBLE] habituation, sensitization, those are a type of memory. You have it in all sensory systems.
We know working memory, which is also transient. It happens in your frontal context, but it also now happens-- there's recent data here at MIT, Andrew Bolton's thesis work, that communicates what's happening in the midbrain tectum, as well. It's only in recent years that these kinds of learning have been found to be associated with anatomical changes, as well.
I'm not going to go through. Just remember, everything is not plastic. The basic pattern we talked about in this class is pretty much the same in all members of a species. Maybe in humans, if you look at the details, you're always going to find differences. Some of those differences are genetic. They run in families. That's another finding out of the Moscow group.
Like the ipsilateral pyramidal tract-- some families have it, some families don't. The massive intermediate, same thing. Some families have it crossing the midline there and another ventricles found there. These are differences. There are sex differences, as well. A lot of that develops postnatally and some of it's genetic, some of it is due to the hormonal effects on development, as we talked about.
Anyway, I talk a little bit about my conclusions about the cortex and the important role of ability we have to anticipate and to plan the major innovation of the neocortex. It's sometimes neglected.
We have acuity, fine acuity, fine motor control, that's very important, too. But it's what came later-- the development of the ability to plan those movements and to use that sensory information for anticipating events-- that's the major innovation. That involves subcortical structures, too, especially structures like the hippocampal formation, as we've talked about.
Then I talk about some other things that I think we need a lot more work on a lot of this, as I've pointed out. I hope you are finishing that last chapter, and I hope the class and the book help you get at least an outline of the whole central nervous system in your minds. In the future, if you encounter more, add to that outline. If you don't have the outline, it's very difficult even to retain what you're learning about the brain.
I found that when I studied with [INAUDIBLE], and I never lost that picture of the brain I developed at that time-- a picture I did not really have at all, or it was a very distorted one before studied it more formally. That's basically what I was trying to do in this book-- to help a lot more people other than my class at MIT do that.
Anyway, you're my keepers. I'm really thankful that you stayed with it. I hope you all do really well on the exam. I'll be happy if you all get As. I posted the review. I know it's a lot of questions. If some of it seems foreign to you or your can't find it, just ask. I will check that forum frequently if any of you actually decide to use it. It actually works. Some years, I get a lot of exchange with students.
But what I will do now-- I do want you to review your mid-term exam, but I'm not going to post any more questions based on the pre-midterm classes. Only the things that occurred after the midterm. But do look at the midterm itself. All I do is I take that review sheet.
I don't always repeat the question exactly because sometimes I think it's a little too vague or something, and I've highlighted words in there that I might ask you to define, just to help you figure out what words might he ask. I've highlighted them there. There could be other words that are involved in the figures, and I've shown you figures I want you to pay special attention to. I do that because I might put a figure on the exam, like I did for the midterm, and you indicate a few of the labels. You all did well on the midterm, so this exam will be very similar.