Flash and JavaScript are required for this feature.
Download the track from iTunes U or the Internet Archive.
Description: This lecture is the third of four on motor systems and brain state, and covers temporal patterns and brain state changes.
Instructor: Gerard E. Schneider
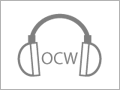
Lec 17: Motor systems and b...
The following content is provided under a Creative Commons license. Your support will help MIT OpenCourseWare continue to offer high quality educational resources for free. To make a donation or view additional materials from hundreds of MIT courses, visit MIT OpenCourseWare at ocw.mit.edu.
PROFESSOR: Let's finish this discussion of the spatial organization of the motor, the descending pathways of motor system. We had talked about these pathways shown in these diagrams, and gone over them a little bit.
I just mentioned the bilateral lesions of the medial pathways. They had to be bilateral. Because those pathways tend to distribute on both sides. So the only way to really eliminate the medial pathways in a way that would really impact function, is to get rid of them on both sides. And they really can't control their body acts well at all.
So they can't write. They can't walk straight. They can't even orient their heads very well. Because the tectum is pretty dominant. And after the cortex is removed, remember that was critical to these experiments. They had to remove the pyramidal tract first. Actually the cortex was intact. It's still connected to the brain stem, except the lower most brain stem.
And that means they have normal eye movements. So you can tell that the monkey knows where he wants to walk. And yet he can't steer himself well, because he doesn't have good control of the axial muscles below his head.
He does-- is able to control this hands though. The only way they could test that was to strap him in a chair so he doesn't have to struggle to try to balance. So then his arms are just dangling and they can present things to him, and he can be able to use his vision to guide his hand to grasp food objects. He does that pretty well.
All right. And then the other lesion that is instead of cutting the medial pathways, they can go in and cut the lateral pathways just on one side. Because they don't tend to distribute bilaterally. And then they got very big differences in control of the two hands. So if they cut the pathways on the left side, he would reach in with his right hand and grasp things, not with single digits, but with using the whole hand. So he could grasp things.
But with this hand he knows exactly what he wants to do. He would reach. The hand just wouldn't grasp. He has control of his arm muscles but he can't do that, so very selective types of paralysis.
Now one of the most interesting parts of that lesion is that if they let the monkey go, so he can run and climb his cage and everything, while he's doing that he's got good control of the hands. So what's going on? When the whole body is involved that system for axial muscle control is really a whole body control system. It controls things like locomotion and climbing. And so in those movements he can control his grasping.
But when you ask him to grasp as an individual movement, separate from other movements, then he can't do it. It's a good lesson on what paralysis means when it's due to a central lesion.
If it's due to a lesion that affects the motor neurons or the axons from motor neurons, then you get a flaccid paralysis. The muscles are just totally relaxed. They're not getting their input anymore. We're just talking about central lesions here.
So those experiments made clear two very different things. One is the spatial layout of the motor pathways, the descending pathways. And the other point was the quantitative dominance of the cortical pathways in monkeys and of course humans. All of those things described in monkeys, you can find clinical cases that match parts of them. But of course, the monkeys gave the advantage of being able to control the lesion much better.
What's the major symptoms of you just cut all these pathways? You can do it-- say someone, a person or a monkey has a transected spinal cord. They die if the lesion is above C4. Why is that? They stop breathing. So you've got to have intact spinal cord down to the fourth cervical level to get control of the diaphragm for breathing.
But what happens to them then? Say around C4 or C5 you get a spinal transection. Say there's an auto accident. The neck is broken. If his breathing survives, he will live. But he becomes what? What's the term? Quadriplegic or the actual grammatically correct word is tetraplegic. But we often mix Latin and Greek in these words. So quadriplegic is actually more commonly used.
So you get a brain that's disconnected from the major motor output below the head, loses control of all four limbs. He does eventually recover reflex control. Of course the spinal reflex pathways are intact. He loses the spinal reflex for a while. That's, as we know, a diaschisic effect. You remove so much excitatory input from those neurons in the reflex pathways that they don't even function. The reflexes don't function. But they come back. And often they become even hyperactive, because of sprouting and denervations, super sensitivity, which are the major reasons you get recovery of the reflexes.
They can think that they want to move. They're able to move in their minds. And we know that, because you can record with imaging methods. And that's what this figure is about. It's in the book. It shows here patients with-- I've forgotten now if one of these is a control. It should be. This should be the control.
But anyway you can see that in the patients they are able to activate parts of the motor cortex. If they're trying to move, you ask them to try to move your right forearm. And the right forearm area of the cortex will increase in its activity. And similarly for other body parts, so they're able to map those areas just by getting the voluntary intention to move. But they don't move, because there's no way for these descending pathways to activate the motor neurons.
So of course this big question remains. Is there any way to get those long pathways to reconnect? And people have worked on various ways to give such people some movement by artificial means. And there are ways that are being developed as you know, and get even some speaking ability in people that have lost these movements. But because of the deterioration of bodily functions that occurs in these kind of people, they usually don't live real long lives after that.
Can you think of a famous actor that this happened to due to a horse accident? Not all that long ago, Christopher Reeve, the guy that played Superman in the long series of Superman movies. So he's very well known, from both films and TV. And they established, the Reeves, established the foundation to support work on regeneration.
I met them. I talked mostly to Christopher Reeve's wife about the-- I was still pretty early on in the research on regeneration. It was after that that we developed these two methods of getting some vision back in animals using the visual systems model, which is easier to work with than the spinal cord. But now it is starting to be done, similar things with spinal cord, as well. Although as I was saying to some of you who talked to me after the class. These things are very slow to move into regular clinical practice.
All right. A couple more topics here on these pathways. First of all, what do we mean by the sensory motor amalgam hypothesis? By amalgam, we mean amalgamation of the primary sensory area and the primary motor area. There are animals, especially the Virginia opossum, when you can't really separate them. That is if you map primary somatosensory cortex, and then you use the method of stimulation, define where the low threshold areas are in the cortex for getting movement, you find it just overlaps with S1, somatosensory area one.
Well how do you know? Is there any purely anatomical method not using stimulation to map those? Then there's a couple other topics here I'll go over.
So that's this question. And basically this is my figure about that. You do it by mapping the projections of the thalamic nuclei that project to this area. So if you take a human, a monkey or another primate like the galago, you'll find that the ventral posterior nucleus that gets the input from spinal thalamic tract from the medial lemniscus, this meta-sensory input, it projects to primary somatosensory cortex. It also projects to a second somatosensory area. But we don't show that here.
And then the nucleus just in front of it, the ventral lateral nucleus, and there's one more-- the ventral anterior nucleus. The ventral lateral nucleus provides the primary input to motor one, motor cortex. It's just in front of the primary somatosensory cortex. And these two areas are quite separate in these large primates, even some of the smaller ones.
But if you look at the rat, you'll find well, it's pretty similar. But there's an area there behind the representation where the two areas do overlap. Meaning that the VP and the VL both project to that area. If you look at the Virginia opossum, they overlap almost completely the area where the ventral posterior nucleus projects, overlaps completely with areas that the ventral lateral nucleus projects.
So there's an amalgamation. They do have a separate ventral posterior nucleus and ventral lateral nucleus. They're segregated-- or no, they're segregated in the thalamus. But in the cortex, it's the same area. They just project in an overlapping way.
In the brush-tailed possum, pretty similar to in some ways to the Virginia opossum, but notice that there's a large area where they are overlapping. But there's also a pretty sizable area where there's a somatosensory area that's not part of the motor cortex. I mean there's a small area of motor cortex that's separate from this somatosensory area. So you get these differences.
So we're pretty sure that the motor cortex, primary motor cortex, evolved out of somatosensory areas, and was probably a separate somatosensory area that became specialized in its descending projections. All the cortical areas have descending projections. And most of them do control movement, even the visual cortex controls movement. It projects directly to the tectum, for example. So it can control eye and head movement, quite apart from-- you don't need motor cortex to get movement from the visual cortex.
Don't think of motor-- motor cortex are not motor neurons. It's just a specialized meta-sensory area that's developed these long direct connections to the spinal cord.
The last thing is this correlation of cortical projections with manual dexterity. And the first thing we look at is just comparing the volume of neocortex in various animals with the projections to the cord. Either how far down in the cord they project, do they go all the way down to the laminae where the motor neurons are, or are they only more dorsal?
And sorry. These are-- layer 10 here is where the-- 10 and 9 are where the motor neurons are. 8, there are the axial neurons. These don't contain any motor neurons at all. And you can see there are animals where there are no cortical axons going to the areas of motor neurons. And there's a number of animals though, where they do go there. They are the ones with greater-- that spinal projection is correlated, as other studies show, with their dexterity.
Let's see this one. Well this one just is another measure of penetration of a cord. But here they're looking at something different, how far down the cord the axons of pyramidal tract go. This is the caudal most cord, [? casidual ?] area. This is the sacral area, lower lumbar. So these are the areas furthest down the cord. So you need the longest axons from cortex.
And you can see if you're an armadillo or a tree shrew, the primal tract never projects to the caudalmost cord. Whereas a few of these animals with the greater dexterity, like humans and monkeys these are other monkeys, it goes all the way down to the [? casidual ?] cord.
And then the correlations with their dexterity, they're not perfect, but if you have some index of measuring how dexterous they are with their hands, you'll find that there is a correlation with the deepest spinal laminae. And if you find the axons terminating in the lowest spinal segment, where do they go? The animals with the greatest dexterity, this group here, are the ones that have the most penetration in the cord.
And in general, the larger the cortex is, as the cortex grows, it becomes not just bigger. The spinal cord doesn't grow at the same rate. So the cord, this neocortex, is relatively much larger than the cord in these animals with the highest dexterity, with humans having the greatest relative size of the neocortex.
And what do we call that rule? That the larger a structure, the more connected it is. It was first enunciated by Deacon. So we call it Deacon's Rule. It's a general rule in comparative neurology that when the structure grows really big, it has more connections. So similarly, the optic tectum becomes very big in some species. And if you look in those species, according to this rule, you're going to find more connections. So when the tectum grew very big, you have more projections even to the thalamus from the tectum and to the cord. Yes?
AUDIENCE: Are there some exceptions to that?
PROFESSOR: Yeah. There are some exceptions. But it's been looked at in-- it's not been looked at for all structures. Like is it-- and it seems to hold when the differences in size are very big. When they're not so big, it's not a perfect correlation. All right. Good question.
And this just shows various studies. I just included a bunch of them here, where they're showing where the corticospinal neurons are in the cortex. They're basically in the motor areas, and the somatosensory areas, both. Somatosensory areas tend not to project to the motor neurons. They project to the dorsal horn, and the motor areas project more to the ventral horn and intermediate layers of the cord.
It looks like animals like rhesus monkey here have more. But that's-- look at the scale. Here are the smaller animals. They all represent one centimeter. So to really compare the rhesus monkey here with one of these, you would have to blow this up so the brain was bigger than the page here, to make the scale match. So you can't really see any indication of Deacon's Rule. But in fact, if you measure, if you count the number of corticospinal neurons, it's very strongly correlated with the total size of the neocortex.
So I have a figure in the book that I didn't put in these slides. But it's fairly recent work that shows that there's really two separate motor regions in the motor cortex. They say the more caudal region is newer. How is it different from the older regions? They're both motor cortex. And you can stimulate both areas and get movement of the same body parts. So what's different?
Did you read it? You've got to read it if you expect to do well. You got to read it more than once, actually. What's the difference? The difference is does the area contain neurons that project direct to motor neurons, rather than just to the interneurons. Remember I said the dominant connection to the cord isn't directly to motor neurons, even for a motor cortex. The dominant connection is to the interneurons that connect to the motor neurons.
But there is an area. It's the more caudal area in the motor-- the strip of motor cortex. We're talking about this strip here. There you see it in the-- so you don't see any indication of that in these pictures, like in the marmoset here. But this would be a somatosensory area. This would be the motor area. There's a more rostral area, and a more caudal area. The more caudal area will contain the ones direct to the motor neurons. And that will vary a bit in size in different species.
AUDIENCE: Is that all animals? Or does that start at--
PROFESSOR: These are maps that show all of the neurons.
AUDIENCE: But that-- so that's in rodents and everything else, or it's not, or it's just in--
PROFESSOR: Yeah. There aren't so many studies that can really-- that have mapped exactly how many neurons there are. It's not easy to study. You see, when you just study projections you can look to see if there are axons that appear to be right in the motor neuron area. And that's about the only way we have of mapping it. And you do find them even in-- in all these species.
All right. But there is-- there appears to be a lot more of them in the large primates. I have a last question here. Does anybody want to try to answer it? What's the highest level of motor control? To answer it, you've got to define what you mean by higher. Perhaps a more interesting question is one-- that's certainly an interesting question. Does movement begin with activity in the motor cortex? Probably not, right? What makes us want to move? Some motivation, right?
AUDIENCE: I think it's in chapter 15 that it's talking about what endogenous [INAUDIBLE].
PROFESSOR: That's one way you could start. So where would you find endogenous activity? Where would you find activity that's varying over time that's not originating outside the organism? Anybody? Probably the activity that corresponds to changing activity, with changing motivational levels.
Yes, we do get a little hungrier when we see good food. That's true. That's a sensory effect. But we also get hunger just because we haven't eaten for a long time. That comes from internal instincts within our bodies. So in the sense it's endogenous to the organism. It doesn't mean there's no stimuli. It's coming from somewhere.
So I answered the question here. I asked that last question. Why is motor cortex become so dominant in humans and in monkeys, and apes? I don't think it's only because of the striatal connections. And all of these areas project heavily to the corpus striatum, important for the learning of habits. I don't think it's because of the direct projections to motor neurons either. I think it's because of its close connections from the prefrontal areas of cortex that contains neurons whose firing is anticipating what's about to happen.
So in the frontal eye fields there, part of the prefrontal cortex, caudal part the prefrontal cortex, you have neurons that fire in anticipation of where you expect something to be. So we move. We say well I move voluntarily to there. Well you were expecting something there. So you move your eyes there. We call it voluntary.
That kind of activity from various parts of the prefrontal cortex reaches motor cortex. That's how it exerts its influence. The prefrontal cortex does not project directly to the spinal cord, and control these movements. It goes through motor cortex. And I think one reason the motor cortex became so dominant is because it's getting that input from the prefrontal areas. And it's most dominant in the animals with a larger prefrontal cortex. Yes?
AUDIENCE: So maybe this is really off track. But did they find motor deficits in the [INAUDIBLE] patients?
PROFESSOR: It's hard to call them motor deficits. But you have incredible changes in the way they behave. Probably the most dramatic are they lose normal inhibitions. It's almost like a release of activity, because there are other influences too on motor cortex. And movement doesn't all go through motor cortex. But what we call voluntary movement does go through motor cortex.
It doesn't originate there. It originates usually in the limbic system, but it's these association areas that include the prefrontal areas, prefrontal association areas, that seem to be where expected things control our movements.
All right. Let's say a little bit about temporal patterns, and about brain states. If we don't have time, we can say a little bit about brain states on Monday. But I'm going to spend a lot of that hour just reviewing to help you get ready for the midterm. But let's just talk briefly about temporal patterns.
And this is an interesting area, because the explanations have been generated as much from theory as from actual experiments. There are experiments related to this. We usually start by talking about rhythmic output, and timing the simplest kind of temporal patterns, it's a rhythmic pattern. And there are various ways that we can talk about how temporal patterns are generated.
We can talk just about reflex types of connections that go from input to output. So the rhythm depends on the inputs, and on the timing of those pathways. It takes time for input to travel, activity to travel through the central nervous system. And then, of course, you can have feedback connections of various sorts that will generate temporal patterns. And you can also have endogenous activity, activity originating in the nervous system and even in single cells.
So first of all, how can the concept of reflexes or more generally stimulus response connections of the sort that underlies fixed action patterns of innate behavior, be used to explain sequences that are longer than a single reflex? If we start with just a single reflex, then we have to talk about conduction time, various things that affect conduction time.
If we talk about beyond the simple reflex, then the only way we can use that model is to talk about some kind of chaining. So let's start by that. And I mentioned the startle reflex. If there's a very loud noise, louder than I could produce here, because I don't have a good amplifier here; you get the sequence of movements that are always the same. The eyes blink first. And then you get contraction of the facial muscles. Then you get neck flexion. Then you get arm flexion.
You see this around explosions. And with a loud explosion you even get leg flexions. But it's always in that sequence. And you can explain that timing by the length of pathways, the fiber size, which makes changes to conduction time. You can explain it by synaptic delays in the pathway. You can explain it by temporal summation times.
And the startle reflex has been a reflex that's been studied in this way. And you get this-- you can explain it just in terms of these timing through the stimulus to response pathway. But when we get to chaining, it's been very difficult to prove there's any real chaining of reflexes. But when it comes to fixed-action patterns, it's much easier. And then we'll talk about the swallowing reflex, so-called swallowing reflex. As it turns out it's not a reflex in the usual sense.
I like Tinbergen's example of fixed action patterns, where you have a whole sequence of movements. But it actually involves two different animals. So for example it starts with a female. The female appears. She's got the swollen belly which is the key stimulus for triggering the male response, which is if he's in a courtship mood, he will start this-- they call it the zigzag dance. A particular way he moves to get the female to approach him. And when she does, then he's swims toward his nest, and she follows him. So that's her next response.
She follows him. And then when he gets to the nest, he adopts a particular posture that causes her to enter the nest. He doesn't enter it first. He changed his posture. She enters the nest. And then the male stimulates her in a very specific way. He trembles, puts his snout at her tail. It causes her to spawn. It's just an innate response. And when she spawns-- actually I left out she swims out of the nest. And as she swims out of the nest, he's right behind her. But he pauses in the nest, and fertilizes the eggs.
They don't have sexual intercourse. This is a way they fertilize. And it's a sequence of fixed-action patterns. Each step is a necessary stimulus for triggering the next step. And in the book I had used that sequence of fixed-action patterns explanation for a behavior, which I've observed many times with Syrian hamsters when they forage for food. It shows the same thing. One fixed-action pattern follows another. Because one fixed-action pattern leads to a stimulus, that then triggers the next fixed-action pattern, and so on. And that ends with they're pushing the seeds out of their pouch back in their nest area. They push it onto a food horn.
So then I ask you here to name a movement pattern in an animal or human that's largely under the control of the hindbrain spinal cord, that is centrally generated once it's triggered. And I want to know how it's different from a reflex, even though it might be called a reflex in clinical neurology.
AUDIENCE: Like swallowing.
PROFESSOR: Like swallowing, exactly. We call it the swallowing reflex. Why do we call it the swallowing reflex? You know how swallowing is triggered? It's triggered by a-- you actually, you think you trigger it voluntarily. But you're not triggering it directly. What you're doing is moving the back of your tongue, so you stimulate the area behind a row of papillae, called the circumvallate papillae on the back of the tongue.
And so a doctor stimulates it if he slides a stick. You can trigger very easily with your finger. What do you get as soon as you get beyond that place on your tongue? You start gagging. What you're doing is trying to swallow. You can't swallow, because your fingers in your throat. And similarly with a stick, so he's simply triggering what he calls the swallowing reflex, or the gag reflex, he will call it.
But that-- we'll come back to the other one there. So it's called reflex swallowing, or reflex deglutition, the name used by neurologists. It actually involves about 20 muscles controlled by neurons all the way from the midbrain to the cervical spinal cord levels, mostly in the hindbrain and upper most spinal cord.
Many experiments have been done to eliminate, after one muscle contracts, to see which stimuli that causes. And you could eliminate all the sources of proprioception. You can even take out some of the muscles involved in the sequence of movements involved in swallowing, and the pattern will continue exactly even when some of the elements are missing.
And those experiments show that it's centrally programmed. Once it's triggered by the key stimulus, it's a centrally programmed movement. It's a fixed-action pattern in the ethological sense. It even has a motivation that builds up. We don't pay a lot of attention to that. But we swallow occasionally to keep our throat lubricated. We don't even think about it. Now that you're thinking about it, you'll probably swallow. I'm doing it myself.
I mentioned here name a movement pattern that's largely under the control of the spinal cord, and completely so in many species. We talked about that one earlier. The pattern-- a pattern that's almost completely determined, probably completely, in the spinal cord. In mammals it requires some external input to get it started, or some input from the brain. But it doesn't require any pattern in those inputs. We're just talking about the movements of locomotion. It's a spinally generated pattern.
Again, it's a fixed-action pattern that's wired in by our genetics. This problem of how patterns are generated was what Karl Ashley called the problem of serial order in behavior. How is a sequence, an ordered sequence-- he used to say how do we do this? And he would move his hand like this. [INAUDIBLE] used to do that. How do we do that?
And he argued, the key part of his argument was that many of our movements are simply too fast for each movement to be separately triggered by reflex. They're too fast. Our reaction times when you measure them for reflexes simply aren't that fast. He talks about the finger strokes of a musician can reach 16 per second. In passages which call for a definite and changing order of successive finger movements, so that has to central generation of movements like that.
And there's not a lot of evidence for various centrally generated patterns, like grooming in mice we mentioned last time, John Fentress experiments. He asked whether, when the mouse starts grooming, well he is generating stimuli, right? So is each stimulus triggering the next movement? So he did things like amputate the arm, and trigger the grooming. The mouse grooms as is if the arm were there. He's not producing any stimuli. You can get rid of any source of the stimulus, and yet the movement goes off in the proper order.
Egg rolling in gulls, it's the same way. They will roll an egg back into the nest, remove the egg in the middle of it, and he keeps doing it. He just does it as if the egg were there. We call those in vacuo movement patterns, movement patterns innate behavior without any stimulus that's any normal stimulus.
And locomotion has been studied a lot. We know that the fin movements in fish are centrally generated. They've even found neurons that are generating those movements. And I mentioned locomotion in mammals. It does require some activation. But it doesn't require patterned activation.
So how are they generated then if it's not by reflexes? And there's basically two kinds of patterns. Either there's got to be feedback in neural circuits, or there's got to be endogenous activity. Or there could be various combinations of those kinds of mechanisms. Those are the kinds we know about.
So with feedback, you can talk about self exciting loops, and I put in this little diagram just to illustrate that. You make certain assumptions about how these neurons are firing, simplifying assumptions. And now you can have a steady input here. It doesn't generate any patterned output. But with one pulse coming in from another source, you could start this feedback circuit, and get the output here to be a series of pulses.
How would you stop it? Well you'd have to either by fatigue or by an inhibitory input, a very simple way you can have a feedback circuit generating a pattern. This system that's been studied the most that is controlled by such circuits is breathing. There's been a number of studies.
There's a guy here at MIT in engineering, named Poon that has studied breathing a lot. It's actually pretty complex. But the basic story always involves these feedback circuits. And there's many examples in our anatomical studies of the brain that show these loops. Anatomists love to talk about loops. That doesn't mean they always know what they do. But there certainly-- the nervous system is full of connections like this.
And then we have endogenous activity. How can a neuron, a single neuron, generate a temporal pattern? This for example, is an example. I took this from Felix Strumwasser's work. He studied big neurons in the abdominal ganglion of Aplysia, because he could identify the same neuron in one animal after the other. And he found that they generated rhythmic activity. Just the-- they had a membrane potential that oscillated, even if you blocked the action potentials, they still generate.
How does it do that? What in the membrane is causing that? They call-- they find specific areas in the membrane they call a pacemaker locus that generates these changes, particular types of molecules. They use energy, and they cause the membrane potential to oscillate. And of course if you don't block the action potentials when the depolarization is enough, you will trigger action potential. So some of those neurons then depolarize enough with every cycle to generate action potentials.
And that's true of some of the very slow oscillators have endogenous activities like this, like the endogenous clock. Single neurons in the suprachiasmatic nucleus will oscillate like this. In this case in the Aplysia abdominal ganglion the rhythm was 40 seconds. But he claims that in that neuron there's also circadian rhythm that changes over 24 hours. I'm not convinced he recorded from them long enough to be sure about that. Because other things could have been changing. But there's definitely good evidence.
[CELL PHONE RINGING]
That just means I have to stop.
AUDIENCE: That's your startle reflex.
PROFESSOR: OK. Yeah, the startle. I'd like you to read the rest of this, and read what I say about overall state of the brain and its control. And we'll have a chance to discuss that a little bit on Monday. And then we'll spend the rest of the time reviewing.