Flash and JavaScript are required for this feature.
Download the track from iTunes U or the Internet Archive.
Description: This lecture completes the development and differentiation of the spinal cord, including CNS structures and the autonomic and enteric nervous systems.
Instructor: Gerard E. Schneider
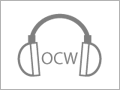
Lec 8: Spinal cord developm...
The following content is provided under a Creative Commons license. Your support will help MIT OpenCourseWare continue to offer high-quality educational resources for free. To make a donation or view additional materials from hundreds of MIT courses, visit MIT OpenCourseWare at ocw.mit.edu.
PROFESSOR: OK, this class, class eight, we're going to talk about what happens to that cord, the development of which we were talking about last time. So we're going to look at the adult spinal cord. And that will lead to a discussion of the autonomic nervous for the last part of class. So we'll look at the different levels, talk about the sensory channels that we've already introduced-- reflex, spinocerebellar, spinothalamic tracts, and another major lemniscal tracts-- and then you'll see the major descending pathways in the cord. At this point, because they originate from above the cord, areas that we've not talked about in detail, this is really just an introduction to those pathways. But the more you're exposed to it, the more likely you are to remember it, and we'll talk about propriospinal fibers.
OK, so first of all, how do the columns of axons of the cord change as you go from one level to the other? It's really a very simple question, and I'm asking why they change. And the answer there is also pretty simple. And then I ask one other thing about those outlines-- what's the lateral horn? So did you try to figure that out? The columns of fibers-- if you compare, say, the cervical level and the lower lumbar level. Yes? Sorry?
AUDIENCE: Is it myelination levels?
PROFESSOR: No, the myelin will be there at all levels.
AUDIENCE: [INAUDIBLE] get smaller.
PROFESSOR: Yeah, the relative size will change. Why?
AUDIENCE: As you go down--
PROFESSOR: Speak up.
AUDIENCE: --count down the more of those ascending tracts are--
PROFESSOR: More of them are terminated as you come down. So there's fewer and fewer axons that haven't terminated yet. And what about the ones going up? More and more are being added-- very straightforward. And do you remember what the lateral horn is? We talk about dorsal horn and ventral horn all the time when we talk about spinal cord.
AUDIENCE: So just for the record, I guess they'll use the [INAUDIBLE]. The ratio is more in favor of the--
PROFESSOR: Look at it here. White matter is in brown here. Do you remember why we call it white matter? When it's unstained in the whole brain-- if you do a dissection or if you do a section, but don't stain it-- the myelin reflects the light more, so it looks white. It reflects all the different colors. And the gray matter here I've shown in a lighter color.
AUDIENCE: I was going to ask-- and this is not really related to this class. But this picture, I [INAUDIBLE] for a long time. So I didn't realize the colors were reversed.
PROFESSOR: This is something I do quite freely, and you have to get used to it. You have to get familiar enough with it so that's never a problem. And you will. When we use different stains, it's going to look different. And almost all stains for axons make them dark. So in any axon stain, it's going to look more like this. So you see, the dorsal columns, the lateral columns, and the ventral columns here, are all much bigger at the top than at the bottom, just for the reasons we were just talking about.
And what about that lateral horn? Just to identify it here, you'll see that there's a dorsal horn and a ventral horn. The ventral horn is pretty wide here because of the cervical enlargement. But right here, you see on the left side, there's a little lateral horn. And here, it's on both sides-- a little bump on the side.
And it's still there in the upper part of the lumbar coordinate, and it disappears. So do you remember what that is? Preganglionic motor neurons of what-- the sympathetic part, the sympathetic nervous system. So we'll talk about that. We'll see it in enlarged figures and so forth, and we'll see exactly what's there.
All right. Now, the thing we didn't talk about here, we talked about gray and white matter. Of course, grey matter, also in unstained sections or in a dissection, it looks grayer because of the cells and fewer myelinated axons.
But look at the ventral horn in particular, and also just the size of the cord. The biggest section is here, not just above it. That's the cervical enlargement. So what's the reason for the cervical enlargement, and then the smaller one down here, the lumbar enlargement? You've got to speak up.
AUDIENCE: Arms and legs.
PROFESSOR: Arms and legs. So that means it should be different in animals without arms and legs, right? And that's true.
So if we take the animal that has the largest legs of any animal that's ever lived, brontosaurus, where we have a complete skeleton-- it's on display still at Yale University, where the curator at Yale discovered it-- look at the lumbar enlargement where they're showing this whole central nervous system here, and compare it to the size of the brain.
You'll see that the lumbar enlargement is bigger than the brain in brontosaurus, because the huge number of motor neurons and interneurons innervating those motor neurons to control these huge legs. And of course, there's also a lot of skin area there, a lot of surface area getting sensory input.
Up above, it's illustrating a turtle spinal cord. Now, what's different about the turtle? Well, he's got a shell. So the body surface, except for his legs, there can't be much innervation. There's not much to respond. He doesn't respond from his shell.
So here, in between the cervical and lumbar enlargements, you do see a lot of motor neurons controlling his limbs. Cervical enlargement would include this one prior to the left. But in between, and below the lumber enlargement, his cord gets very small. It's still needed, because it is innervating various bodily organs at those levels too.
So who is this guy, Bror Rexed? Notice the pronunciation-- Rex-ed. Bror Rexed. He added something to the way we talk about the spinal cord, especially when we're doing cyto and fiber architecture of the cord. What was it he added?
Layers. He studied the spinal cord of various animals as well as human and published a series of papers and was consistently able to define the same layers. And this is just an example where you see the Nissl-stained section. This is the seventh cervical segment. So these are motor neurons innervating the [INAUDIBLE], so the arms and hands. And you can see a lot of motor neurons.
But they're in two groups-- the lateral group that's actually got several different clumps there, he's outlined it here. It's layer nine. And this one he called layer eight in here. These are so much layers as groupings. These are the motor neurons innervating the body axons.
And then all of the interneurons that tend to be premotor, they contact the motor neurons, most of them. That's layer seven. He does have a layer 12, this area right around the ventricle there. Notice how relatively small the ventricle looks now. It's not because it got smaller since the embryo. It's that everything else got so much bigger. And then you see his other layers.
And the dorsal horn in particular, it's very easy to identify the layers. So if I ask you to describe some differences in cytoarchitecture of the dorsal and ventral horns, you see, you would mention the motor neurons. They're the largest and usually deep-staining neurons, the motor neurons in the ventral horn.
And the dorsal horn has the most clearly defined layers. You can see it best in a fiber stain, like here. Here's a human lumbar level, and you can see. Very light myelin there in layer three. So you see layers one, two, and three always stand out. The layers are easy to identify.
There's one of a rat. Because of myelinated axons you can hardly see-- layer two doesn't look very light. And also I've done it with a very high contrast here. But the upper layers are very, very white.
All right. So myelin has been called a crucial vertebrate innovation. That was a quote from Allman's book. So what is it that made myelin such a crucial innovation of the vertebrates? It's not found in invertebrates, and it's actually not found in the most primitive vertebrates, the jawless vertebrates, the hagfish and lamprey. But it is found in all the rest of them.
So why was it so important? Yes?
AUDIENCE: Does it help with increasing the speed?
PROFESSOR: It allows the increase in speed of the action potential without increasing the diameter so much. Invertebrates do have pretty fast conduction, like in the squid. They're a pretty big animal. And to get fast enough conduction for their escape response, they've developed these giant axons. They're, like, a millimeter in diameter.
But that is a lot of cell to maintain. If you want very many axons to get finer control, that wouldn't be very good for the vertebrates, the larger animals. Can you imagine a whale trying to control his muscles with any speed at all if he didn't have myelin? His movements would be incredibly slow. His reaction time would be very slow. Yeah?
AUDIENCE: So myelin is like [INAUDIBLE], right? But I wasn't sure how, actually, it helps [INAUDIBLE].
PROFESSOR: OK. If you want to search in my book, just search-- you've got the PDF files, so you can search under myelin. And you can look for saltatory conduction. Saltatory means jumping. I did talk about this briefly in class before. The action potential jumps from node to node. A node is where there's no myelin. The myelin is never continuous. And where it is present, it insulates. So you can't get the ion hook through the membrane. So that means all the ions have to flow at those nodes. They're close enough together.
So the detrimental conduction down in the membrane, which is very, very fast, is strong enough, it won't decay enough, that it will reach the next node and trigger the next action potential there. So it literally goes-- you only get the action potential at the nodes.
AUDIENCE: So it's kind of like dominoes?
PROFESSOR: Yeah, exactly. It's like a series of dominoes falling. And of course, if something happens, if you get an area where the node is too far, the action potential will just stop. And this does happen sometimes, especially in certain pathologies.
So one more question here. How does the spinal cord of humans differ from the spinal cords of other mammals? How are humans different? What do we do better than other animals?
Well, for one thing, this kind of thing. That requires a lot of dexterity, tremendous control of these fingers. That requires a lot of axons coming from the brain that controls motor neurons. So that's going to mean a lot of descending axons.
So the white matter, with those descending myelinated axons, is going to be big. Another question?
AUDIENCE: Do other animals have something to the equivalent of our [INAUDIBLE]?
PROFESSOR: Yeah, all the animals have these same parts. You know what the cauda equina is? They're roots. They're dorsal and ventral roots below the level of the spinal cord, you see, because our spinal cord doesn't grow as fast as the rest of our body does. So the spinal cord ends at the small of your back here-- fairly high up.
But your spinal column, the bony spinal column, continues further down. And in that spinal column, you have the dorsal and ventral roots for the caudalmost part of the cord. That will be similar in animals. It's very similar in other primates and humans. But it's present in other animals too.
The biggest difference is in sensation and dexterity. To get that very-- we have a dense innervation of these fingertips. Now, for the spider monkey, he's got some dense innervation of his tail too, and he's very dexterous with his tail. He uses it a lot. So he also has a lot of fibers for that. So that means the dorsal roots, and that leads them to the dorsal columns for the fast-conducting pathway that's also bigger in humans.
AUDIENCE: What about our ability to stand upright?
PROFESSOR: The ability to stand upright, that required evolution of special feet, special vestibular control, vestibular reflexes, and somatosensory reflexes from the soles of our feet. So people that wear thick prosthetics in their feet don't lose some of that. But we compensate very well for it in various ways. So don't worry about it if you do.
All right, so just note here, I'm going to compare human and rat. Look at the lateral column here. You can see it in the drawing more easily. And now look at it in the rat. There it is in human.
Why is this so much bigger? Especially because of the descending axons, coming from the neocortex. The dorsal columns are also bigger, but this is lumbar level, where the difference is great. But you can see this is not as big as this. But you notice that difference very much more at the cervical level.
All right. So let's look at the sensory channels now. We'll talk about reflexes, the spinoreticular, spinothalamic tracts, spinocerebellar, and how the dorsal column axons get started. We'll start with this question-- where do the largest axons in the dorsal roots originate?
So now we're going to start looking at cross-sections of the cord. We're going to look at the axons coming in and the circuits they form. But where do the largest axons arise? I talked about it twice in the class already, but you may not remember. That's why I repeat myself a lot-- because I know that when you're learning neuroanatomy, you're hit with a lot of these things. And you have to hear it again and again.
So I often tell you, just understand me. Don't worry about writing it all down. Just understand me. If you understand and you keep encountering it, you just start to remember. That's also why it's useful to come to class-- those of you who are not here-- because going over it is actually very helpful for learning. Some of you, in some of your classes, can read it once and you'll be fine. If you do that in neuroanatomy, you won't be fine, unless you are-- maybe Sheldon could do it. All right. In case you don't know what I'm talking about, you don't want watch TV in the evenings, The Big Bang Theory.
OK. Here is a section of the cord, and this is from a medical book. And it's illustrating different sizes of axons in the dorsal root. It just gives one example of the largest axons here. They're called A alpha axons. Do you remember where they're coming from? They're coming from the stretch receptors in muscles. Where are the stretch receptors the densest? These muscles doing this. Any place we have a really good control-- where would another good example be?
AUDIENCE: The tongue?
PROFESSOR: The tongue, exactly. We have to have extremely precise control of the tongue in order to talk. And we're also pretty good at eating. All right, so here they are, big axons. And there's the branch going into the dorsal column. And here it comes into the cord. And it's terminating here in layer six, including some very-- those are ones destined for the cerebellum, and we'll see that in a minute.
But the most important thing here is they go directly to motor neurons. Some of them contact the motor neurons, so it's a monosynaptic reflex, the stretch reflex. Whenever a muscle stretches, it triggers those receptors, and it tends to cause the same muscle to contract.
You say, well, that's counterproductive. No, it isn't. It's a perfect way to maintain good muscle tone so you're ready to move. If you don't have good stretch reflexes, so you don't have some tension in those muscles, you can't respond quickly.
And if you wonder, when you're moving around rapidly, if I'm moving my arm like this, you might be surprised if you do this. Feel the muscle on the other side. It should just be the biceps here, right? But you'll see that the triceps contract also when I do that. Any rapid movement, that's true.
All right, so what else did I ask you? We already talked about that.
Describe the axons and connections of a withdrawal reflex-- the flexion reflex. This came up before. And then describe the origins of the spinothalamic tract within the spinal cord. And we'll contrast that with the spinoreticular tract.
So first of all, what was the characteristic of the withdrawal reflex? How is it different from the stretch reflex? What would be the difference? The first synapse would not be on the motor neuron. It always involves at least two synapses in the cord.
So here, in this little sketch, I show, first of all, they're not the largest axons, but they're axons that terminate in the dorsal horn or in the intermediate layer. And those cells, like this one, can contact a motor neuron. That would be the shortest pathway to a flexor muscle to get the withdrawal reflex. The fibers carrying that input tend to be small, carrying input from pain sensations. It also happens with extreme pressure and so forth.
Note, there are similar connections here. I show same axon with a branch that gives rise to a flexor reflex. And there's a branch here contacting a neuron that sends its axon across to the other side, becomes a spinothalamic tract axon. That's the typical origin of the spinothalamic tract. Remember, the spinothalamic, no more than 20% of the axons, even in the higher primates, actually reach the thalamus. They go to the reticular formation, the hindbrain, the midbrain, they go to the older parts of the thalamus, and then some of them go to the new parts of the thalamus that project the neocortex.
So how is the spinal reticular pathway different? We call this spinothalamic, even though it does go to reticular formation too. But when we call it spinoreticular, we're talking about an even older pathway before there was any crossing. So a pathway you find in the most ancient of the vertebrates, like hagfish and lamprey. And even amphioxus has pathways like the spinal reticular. There they are.
They come from neurons here to get input in the dorsal roots. The axon enters the lateral columns on the same site, and the axons ascend. Some of them actually terminate in the spinal cord, but many of them are reaching the brain. They're called spinoreticular because they terminate mainly in reticular formation at all levels of the brain stem. The longest ones do get into the thalamus, but most of them terminate in the older parts of the thalamus and subthalamus. That is, the parts that don't project the neocortex, or at least that's not their only projection.
This is just for those of you who are curious what you might encounter in medical school. You will get pictures like this where they show in blue here, spinoreticular pathways in a bunch of sections of the human brain. This is midbrain up here, caudal midbrain here, and there's the pons in human.
You can see how much I've simplified when I present this introduction to neuroanatomy. Understanding that concepts is the most important thing. Once you understand them well and get that organization in your mind, then figures like this won't confuse you so much. And they do show-- some of the axons right here-- going above the midbrain to intralaminar thalamic nuclei in hypothalamus. We haven't talked about what are the intralaminar thalamic nuclei, but that's a name for the older parts of the thalamus, parts that survived complete removal of the neocortex.
All right, so where do the longest axons of the dorsal columns originate? And where do they terminate? We introduced the dorsal column, medial lemniscus pathway, less time. It's different from the spinothalamic tract, but like the spinothalamic tract, it's a pathway that carries input to the opposite side of the forebrain.
So where do the longest axons of that pathway originate? Well, we know where they terminate. The dorsal columns are primary sensory axons. They're these axons we're talking about, up here, the dorsal columns. And as I show here, the primary sensory axon doesn't terminate on anything there. It just turns rostrally. It often has a caudal branch too, but we're concerned about the rostral branch. The primary sensory axon goes all the way up to the top of the spinal cord, to the dorsal column nuclei.
They have names, dorsal column nuclei. I don't care if you even remember that right now. They're called the slender nucleus and the wedge-shaped nucleus. The gracile and cuneate nuclei-- nucleus gracillus, nucleus cuneatus. Whenever you learn medical school anatomy, you learn these names.
But remember them just as the dorsal column nuclei for now, and they are the secondary sensory neurons. So where would the longest axons originate? We know the nuclei are way up at the top of the spinal cord. So the longest axons originate from the most caudal part of the body. That's not the feet.
Remember, the dermatome map. They originate in the caudalmost dermatomes-- right around your tailbone in the [INAUDIBLE] of your tail. That's where they originate, and they go all the way up to the top of the spinal cord. And they're primary sensory axons-- rapidly conducting. They tend to be larger than spinothalamic tract axons, so they conduct more rapidly.
Those longest axons terminate in the most medial nuclei. It's characteristic of dorsal columns that the axons of the dorsal roots enter, join the dorsal columns from lateral side. So the further up you go, the more lateral the axons are. And the ones that originate more caudally are more medial.
And when you get, then, to the dorsal column nuclei, they have an organization there. It's quite topographic. And then once you understand that, you can answer this question. Explain how there's an organized representation of the surface of the entire body in cell groups at the dorsalmost end of the spinal cord, those dorsal column nuclei.
But wait a minute-- if it's the entire body, it has to include the face and head, right? So why is that represented up there? I say the whole body.
So here's my picture of it. Here's the dorsal columns. And as you get up to the top of the cord, cells appear, right among those axons of the dorsal columns. One group here, medially, that's the more slender nucleus. It represents the lower part of the body, the tail region, the lower legs. And then the nucleus cuneatus tends to be larger because the forelimbs-- certainly in humans, especially, the arms and hands-- are highly innervated. So you have many more axons terminating. That's where the upper body is represented.
But out here in the dorsal columns of the spinal cord, the face is represented. That seems really odd when you first encounter it, but it's because axons that come in through the trigeminal nerve, some of them descend and run along, through the hindbrain and into the top of the spinal cord. They're found right here at this level, the upper cervical cord. They are terminating in those cells there of the dorsal columns representing the face. The sensation that they are responsible for, those ones that descent that far, are pain and temperature.
And that's why a surgical operation for very difficult-to-control sometimes consists in going to the very top of the spinal cord. It's dangerous surgery because you can't do much extra damage. They will actually cut these axons to reduce the pain. The only trouble is, surgery to control pain is almost never permanently successful. Pain always tends to come back, and there's various reasons for that, but it's still an area of active research-- representation of pain in the body.
OK, so the entire body here represented in a topographic way, from the caudal end, medially, to the face area laterally. At the level of the dorsal column nuclei. So now let's talk about spinocerebellar.
The source of axons terminating in this column of cells we call Clark's column-- sometimes just called nucleus dorsalis, the spinal cord-- from the lower limbs. And the descending fiber tract that originates in Clark's column is one of the spinocerebellar tracts. This one's called the dorsal spinocerebellar tract. It travels like this.
So here we are, axons coming in, terminating in nucleus dorsalis, or Clark's column, right there. Those are lateral horns, so you know you're in most likely the thoracic spinal cord. It could be even upper lumbar. And the cells there send their axon into the more dorsal parts of the lateral column and then turn rostrally. And they ascend all the way to the cerebellum.
That's one of two or three pathways, depending on the animal. But most of them have at this level two different pathways-- an uncrossed pathways like this and a crossed pathway where the axons crossover and are much more like a spinothalamic tract, but also go to the cerebellum.
So where are they originating? What kind of information is important for the cerebellum? They carry information on joint movements, primarily from the lower limbs and the trunk. So whenever you're moving, you are generating sensations that you can't-- we don't have this much conscious sensation, although we do have some sense of where our arm is positioned because we do some of that, but it doesn't go directly to the cerebellum. It goes also to the cortex. But that's the dorsal spinocerebellar tract.
OK, another group of axons. Propriospinal-- it literally means proper to the spinal cord. In other words, they never leave the spinal cord. There's a lot of axons like that.
So for example, if we transect the spinal cord in an animal, keep him alive-- if we make the cut low enough, he will live even without artificial respiration. Below the level of C4, you'll still be able to breathe. But if we really completely transected the spinal cord, the movements of the entire body caudal to that level will have to be controlled only by the spinal cord.
And after a period of recovery, you can get different gaits. You can get locomotor patterns because of propriospinal connections, which are responsible for fixed action patterns-- that is, they're under genetic control.
Let's look at this picture on the left side, the orange ones, this sketch propriospinal axons, they tend to run in the columns of axons nearest the gray matter. And I'm showing cells in the gray matter sending axons into that goop. They go to another level and then they terminate. So it goes from one part, mostly intersegmental axons, passing from one segment to the other.
And then I'm asking about the descending axons that influence movements of the limbs and other movements. Those movements are heavily influenced by pathways from the brain. And at this point, just read the names, get an idea of where they're coming from. The big ones here in human, and another primates-- especially the large primates-- coming from the neocortex.
This is the position of the corticospinal tract, the blue dots here, in humans and monkeys and apes. And I'm showing how the axons tend to distribute throughout the spinal gray matter. I show another pathway there that has a fairly similar trajectory coming from the midbrain.
Call it the rubrospinal tract. "Ruber" means red, and they come from the red nucleus in the midbrain. We'll be talking about that when we talk about midbrain. They have a little bit more limited distribution, and they terminate mainly in the enlargements, not in between the enlargements, whereas corticospinal tends to terminate on everything.
And then-- oh, I should mention one more thing about the corticospinal. It's not always in the lateral columns. When you're a comparative anatomist like I am, you really have to pay attention to this, especially if you're using the animals to study the pathways. Like, I did a study with a student of the corticospinal pathways in the hamster and their role in the behavior of the animal.
Well, it turns out we wanted to cut the pathway. They don't travel here. They travel in the ventral part of the dorsal columns. That's true of the rat too. It's true of the tree shrew. It's true of many animals. For a while, we thought the tree shrew was a primitive primate until somebody looked at the corticospinal pathways and found they weren't in the position of primates. They were in the position of insectivores and rodents-- here.
OK. The ventral columns here, which in relative terms are similar throughout most mammals, they contain the axons that control the body axis, of posture, vestibular reflexes, writing reflexes, also orienting movements if they're very far rostral.
So I've listed the main ones here-- reticulospinal. A lot of axons in the reticular formation that control fixed action patterns. They send their axons-- most of those involve axial muscle control, or at least whole body movements. Some of them might go to the motor neurons controlling the limbs as well. But always, whole body movements or axial muscles. And then vestibulospinal-- fastigiospinal, sorry. But nucleus fastigius is a deep nucleus of the cerebellum. So you can think of it at this point as just cerebellospinal.
And then tectospinal, from the tectum of the midbrain, for turning movements. They only go to the cervical part of the cord. There are pustule adjustments whenever the animal makes a head movement like that, but that involves other neurons, usually from the reticular formation, reticulospinal.
All right. And here I just have the same picture, and I'm just showing that these are almost all ascending axons in humans and other primates in the dorsal columns. If you're a rat or a tree shrew, then you'll find some corticospinals here. But these are otherwise in us all ascending axons.
The lateral columns are ascending and descending. And by the way, I'm not including here propriospinal. They're always both ascending and descending. They just don't leave the spinal cord. And in the ventral columns here, they're all descending axons, almost all.
All right. We have only a little bit of time to introduce the autonomic nervous system, the peripheral nervous system components that innervates the glands and smooth muscle, and also the cardiac muscle, which is another type of striated muscle. But it's innervated by the autonomic nervous system. And so I'm giving you the answer or talking about-- sometimes it's called visceral nervous system. It innervates the viscera. But we call it autonomic. And it contains these two components, as most of you already know-- sympathetic and parasympathetic. There's a third component that we can think of as more like parasympathetic, but we mention that as well.
We want to contrast the functions. How do people usually summarize the functions, functional differences between sympathetic and parasympathetic nervous system? Take the heart, for example. Sympathetic nervous system, what does it do to the heart? It speeds it up. So parasympathetic slows it down.
What about the gut, peristalsis and all that? Most of that's controlled locally, but it's also affected by autonomic nervous system. Sympathetic nervous system tends to stop that and tends to increase blood flow to the muscles. We call the sympathetic nervous system the fight-or-flight system, because those are two situations where the sympathetic nervous system becomes totally dominant.
The autonomic system is always active at rest, and it's the normal functions of organs controlled by the parasympathetic nervous system, not involved in emergencies and fighting and fleeing. This table, you should read. Get familiar with-- I'm just listing some of the major organs, describe some of the things we've just talked about.
There are some areas which are a little harder to figure out, just from that fight-or-flight analogy like the sex organs. You can read that. That involves also both systems. Almost all the bodily organs are innervated by both sympathetic and parasympathetic.
And the innervation is a little bit different from the innervation of striated muscles. If you look at the motor system most broadly-- this was from Swanson-- we know striated muscles are innervated. This is the somatic muscles, innervated synaptically, local synapses that act locally. But the autonomic is innervated differently. We call it paracrine innervation.
The neurotransmitter is released, and it's sort of spritzed out and affects a bunch of nearby cells. So you don't have the tight synaptic context that striated muscles have with the muscle [? in ?] [? place. ?] And then of course you have the neuroendocrine system, which is really a third motor system where the secretion is in the bloodstream. So then it's like apocrine to an extreme. The whole body is affected.
Now, the two divisions, sympathetic and parasympathetic, they both have apocrine innervation of the end organ. But the innervation is through ganglion cells in the periphery. So here the dashed line represents the division between CNS here and peripheral nervous system here.
But in each case, sympathetic and parasympathetic, there's a ganglion cell. They're located near the CNS if they're sympathetic nervous system. And they're located near the end organ in the case of the parasympathetic nervous system. So just one example here, my schematic view, I've only showed the innervation of the iris of the eye and the gut here. And here, I've just called the pelvic organs. I've not drawn any pictures.
So we just take these three. Look at the iris. Sympathetic nervous system, the ganglion cells are always near the spinal cord. They extend from the superior cervical ganglion in the neck all the way down to below the cord. They're always innervated by axons coming from somewhere between T1 and L2 and 3. That's where the preganglionic motor neurons are.
But this rostralmost ganglion here, superior cervical ganglion, sends axons into the head region. And some of them go directly to the iris. So when we get pupillary dilation, when our sympathetic nervous system is activated, like if we're angry or we're frightened, pupils dilate, bring in more light, reduce our resolution a bit.
That comes from here. The parasympathetic system, that's the craniosacral system. It comes from the third nerve nucleus here. It's a little sub-component of the third nerve nucleus that's parasympathetic. It's called the Edinger-Westphal nucleus. So I've written there, the EW nucleus.
It's in the midbrain. The axons go out. They don't go directly to the iris. They go to a ganglion behind the eye-- the ciliary ganglion. And the axons of those ciliary ganglion cells, they're the ones that innervate the iris. And they use different neurotransmitters. The neurotransmitter in the ganglion is the same. It's always acetylcholine. And then the purple cell, the final cell there, is acetylcholine again for the parasympathetic, but it's norepinephrine for the sympathetic.
And we're going to stop here. And I will put all these slides online. I might review a few of them at the beginning of the next class.