Flash and JavaScript are required for this feature.
Download the track from iTunes U or the Internet Archive.
Lecturer: Prof. Tyler Jacks
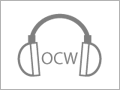
9: Genetics 4
OK. We are wrapping up our segment on genetics today, Genetics 4. We're going to talk about human disease genetics, modes of inheritance for human diseased genes. And then we'll transition next time into molecular biology, which is the next blank square here. And then recombinant DNA cell biology and beyond. I wanted to start today by clearing up a couple of things. Firstly, the term F1, in the last lecture I gave you a list of terms, and I used this definition of the first filial generation, the F1 generation as the product of crosses between two homozygous individuals, homozygous for different alleles such that the offspring were always heterozygous, big A, little A at that particular gene. Well, it turns out that some people use the term F1 to refer to the offspring of any cross. So you have the parents and you have the F1s regardless of the genotype of the parents. So that's a looser definition of the term F1. And it's been used in Section and so on, so I didn't want to confuse you. The strict definition is the one I told you, but don't get hung up on that. We're always going to give you the relevant genotypes so you'll be able to figure out what the genotypes of the offspring are. Also, near the end of the last lecture, I talked about crosses involving linked genes. If you remember the Y and D gene controlling color and density, I think, of peas. And I told you that they were linked, and we carried out a test cross. And we then worked out what the percentages of the different phenotypes and genotypes would be. Well, in that example, I assumed that the Y allele and the D allele were together on the same chromosome. But importantly if you're just given the genotype as it's written here, you actually cannot know that. The big Y might be on the opposite chromosome from the big D. So to avoid confusion about that sort of thing, in future examples and in problems, problems, that is test questions, we'll always show you the chromosomes so you'll know that the genes are together on the same chromosome or on opposite. Yeah. And in the absence of this, if the question is to tell us what the alleles must look like and they give you the phenotype, you have to conclude the genotype. If the question is tell us what the alleles must look like then we're not going to give you the answer, but in other situations where there's ambiguity about what the alignment might be, we'll tell you what that is. OK? We also had a question from a student, actually an emailed question, those are also welcome, email question from a student. It was good question because in our discussion of dominance and recessiveness, we really haven't dealt with the molecular nature of that. And that was this individual's interest. Why is an allele dominant over another one? Why is a trait dominant over another one? And I gave an example to him, which I will give to you, which I think covers most of the examples that we've been discussing in class. And it also is an opportunity for me to reinforce the notions related to chromosomes, genes, DNA and proteins, because apparently there are some of you who are still a little fuzzy on those relationships. So let's imagine a gene which is present on a chromosome. Chromosome shown here. Gene shown here. And it's the S gene that we've talked about before that controls smooth versus wrinkled pea texture. So here's the S gene. It's made up of DNA. And based on the sequence of that DNA within the gene, therein lies the information to produce a protein. And we're going to call this protein, in our example, this is a hypothetical example, the starch synthase protein. It's an enzyme that controls a reaction, that catalyzes a reaction from some sugar substrate into some starch. And if you make enough of that starch product then you have a smooth shape. OK? This enzyme controls shape because it produces this product which is involved in the shape of a pea. OK? So this is the normal situation. The big S allele produces a functional starch synthase enzyme which produces enough product to give the pea its smooth shape. In this example, the little S allele, which is the recessive one, has a mutation within the coding sequence of the gene. We won't talk about the nature of that mutation just yet, what it is, why it causes what it does because you're going to get that in the next segment. Just suffice to say that it's a mutation, an alteration of the DNA such that the protein that's produced from this allele is nonfunctional. You might be able to see I've inserted a little X there. It's actually quite little and invisible on your handout, but you might want to just circle it if you look carefully. There's a little X there which is the result of this mutation. And that X causes the protein to be nonfunctional. The enzyme now does not catalyze the production of this starch product, so no starch product is produced. If you don't make the starch product you don't have a smooth shape, you have a wrinkled shape. OK? If the genotype of the pea is little S, little S then none of this enzyme is produced such that none of the starch product is produced such that the pea is wrinkled. OK? Now what happens if you're big S, little S, the heterozygote? Well, for many biochemical reactions, having just one copy of the gene that encodes the functional enzyme is enough. For many biochemical reactions that's true. And so in a situation where big S is dominant over little S, we assume that having one copy of big S makes enough of the starch synthase protein to make enough of that starch product to give the pea its smooth shape. OK? So that's a simple example of why big S is dominant over little S, why you only see the phenotype associated with the little S allele, the wrinkled phenotype when you have two copies of the little S allele. OK? So I hope that helps clarify the situation for you and actually is useful as we talk about human disease genes as well. So this is a slide from your book which allows us to transition from concepts of inheritance to real-life stuff. Genes that regulate how your body functions normally or in response to various environmental stresses. We're now in an era where we can relatively easily figure out whether a disease has a genetic component by looking at families that might have that disease, and based on that information and using mapping techniques like I described to you last time, we can isolate where that gene might lie on all of your chromosomes. And then using molecular techniques, which we'll talk about in future lectures, we can actually isolate that gene, determine its sequence, and based on that produce lots of various valuable things like better ways to diagnose the disease, better ways to understand how the disease process takes place such that we can then perhaps prevent the disease from occurring in the first place or treating it more effectively by replacing the gene with a new copy or producing a drug that can replace the gene in other ways. So this is what we're after. So we need to understand diseased genes and how they behave in such affected families. So there are a number of diseases which have a genetic component. And these diseases have various modes of inheritance. Some of them are autosomal dominant. The diseased gene is dominant over the wild type gene, and I'll give you examples of that. And the term autosomal means that it's not sex linked. That is the disease gene is carried on chromosomes 1 through 22, one of the those chromosomes, not on the X or the Y. It doesn't matter if your father, whether the disease gene is coming from a male or a female, passed on to a daughter or a son. There's no sex linkage for these autosomal dominant diseases. There another class of genes, disease genes which follow autosomal recessive inheritance patterns. Here the disease gene is recessive to wild type. You only see the disease phenotype when you're homozygous for the mutant allele. And, again, autosomal because it's not sex linked. There are X linked diseases which are dominant. In this case, the disease gene is on the X chromosome. There are also X linked diseases that are recessive. There are very few, but for the sake of completeness, Y linked diseases. But there are so few that we're actually not going to talk about them at all in this class. And, finally, there's a class of diseases that we're also not going to talk about but get inherited not from the genes that are in the nucleus of the cell along your chromosomes but rather get inherited from the mitochondria. And since we haven't talked about mitochondria with you really at all we're not going to expect you to know about those diseases, but just have in the back of your minds that those are relatively important. Autosomal dominant diseases are not terribly common. There are about 200 known. Autosomal recessive diseases are actually much more common, though not very frequent in the population. There are about 2, 00 of these known. And together I would estimate there are about 25 sex linked diseases. So we've used the term autosomal dominant, autosomal recessive, sex linked. What does this really look like in terms of the genes and the alleles? Well, just as in the case of peas, a dominant disease allele will cause disease regardless of the nature of the other allele. It's dominant over the normal common version of the gene. So if we call this allele the disease allele of some gene -- -- and this allele the commonly found on in the population, and we refer to these often as the wild type -- -- in an individual who has this genotype for a dominant disease gene, will they develop disease? Yes, because the disease allele is dominant over the normal copy of the gene. So these individuals will develop disease. For recessive disease alleles you need to have both copies, both alleles be mutant in order to manifest the disease. So here's another gene, which we'll call the H gene, which has a disease allele. This individual is homozygous for the disease allele. Will this individual develop disease? There might be other people in the population who are heterozygous for that allele and have a wild type allele on the other chromosome. Will they develop disease? No. These individuals are called heterozygous carriers. They carry the disease allele, but because they also carry a wild type allele they don't develop the disease. They're normal. They're normal in the sense of their phenotype. They have an abnormal genotype in the sense that they have a disease allele, but they're normal in the sense of their genotype, phenotype. Now, for X linked genes, sorry, before I do that let me -- Let me review for you sex determination. We talked about this briefly. In order to understand the inheritance pattern for X linked genes you need to remember this. That males of course have one X chromosome and one Y chromosome. Females have two X chromosomes. If you think of a Punnett Square related to the inheritance of chromosomes males will pass along an X or a Y at 50% each, females will pass along one of their two Xs. Will produce an equal number of males and females from such crosses. But it's important to think about where the X chromosomes come from, specifically males and the Y chromosomes. Males transmit their only Y, their only Y to all of their sons. If you're the son then you've gotten your father's only Y. Males transmit their only X chromosome to all of their daughters. If you're the daughter, when you're the daughter of a male, you have inherited his X chromosome. OK? Females transmit either X to each daughter or son. In the case of the female X, you can be a male who got this X chromosome or a male who got this X chromosome. You can be a female who got this X chromosome or a female who got this X chromosome. And that's important for understanding the disease genetics. So let's imagine a dominant disease involving the X chromosome. A gene, we'll call it Q. Males only have one X chromosome. They only have one X chromosome, therefore if they carry the disease gene they're going to get disease. Females have two X chromosomes. They might carry the disease allele on one but a normal copy on the other. In this scenario, will they develop disease? Yes, because it's a dominant disease allele. For recessive X linked disease, once again, males only have one X. Are they going to get disease? Yes. Females have two X chromosomes. If they carry a disease allele on one they are likely to carry a wild type copy on the other. Are they going to get disease? No. They're going to be heterozygous carriers. And you'll see how this plays out towards the end of the lecture. OK. So let's look at some examples. We're going to start with recessive diseases first, recessive autosomal diseases first. And one you've already seen before, and that's PKU, phenylketonuria. If you recall, this is a disease which is associated with failure to make an enzyme that converts phenylalanine to tyrosine. It's an enzyme called phenylalanine hydroxylase. If you don't have that enzyme then you produce too much of this byproduct, phenylpyruvic acid which is toxic and leads to brain damage and retardation. This can be avoided, the disease can be avoided by limiting your amount of dietary phenylalanine. And that's why such patients are not supposed to have Equal, as we discussed before. The disease, PKU, is autosomal recessive. The incidence is rare, about one in 10,000 to 15,000 individuals have PKU. As I talked to you about last time, the mutant allele has a single amino acid chain change in the active site of this enzyme which changes an arginine residue to a tryptophan residue. And that makes the enzyme inactive. This is an autosomal recessive disease. So if you have one mutant allele and one normal copy of the phenylalanine hydroxylase gene, do you have the disease? No. You would be a carrier but you would not have the disease symptoms. Because again, as in the other hypothetical example, most of the time, if you have a single normal copy of an enzyme that carries out some biochemical reaction that's enough and your phenotype is normal. Here's another common example, cystic fibrosis. This is actually a more common disease. It affects about one in 2, 00 to 3,000 individuals. That's not an insignificant number. So there are a lot of people with cystic fibrosis in this country. Again, it's autosomal recessive. Patients present with the disease at birth. They have problems both in their intestinal system as well as in their lungs. It tends to be the lung symptoms that ultimately kills the patient due to inability to breathe properly, but actually more importantly persistent infections which ultimately are so severe that they lead to death. And these patients tend to die within the first two to three decades of life. The gene encoded by the disease, the gene responsible for this disease is a chloride channel. When it functions properly it allows chloride ions to move through, and this achieves a proper water balance inside the cells. When the disease allele is present in both copies, this channel is not formed properly, and therefore the water balance in such cells is not correct and it leads to the build up of this kind of mucousy sticky substance both in the lungs and in the intestines. These individuals, as you can see, have a very hard time breathing, and they have to get this mucous cleared from their lungs periodically. And they also often have to have respirators to allow them to breath. OK. So that's some examples of autosomal recessive diseases. Let's think about the genetics. And we'll talk about CF specifically. Let's imagine the disease allele of CF. We'll call it CFD. There are actually many different disease alleles for this disease, but we'll just lump them together. Here are two individuals with this genotype. Do these individuals manifest the disease? Yes or no? Do they manifest the disease? No, because this is an autosomal recessive disease and they have a wild type copy. But if we think about the offspring that they could produce, this individual will produce gametes that carry the D allele and the wild type allele, likewise this individual, the D allele and the wild type allele, such that the offspring will either be DD, wild type D, wild type, wild type or wild type, D. Right? So you'll have one wild type, wild type individual produced out of such a cross. And this individual now has no disease allele present. This individual is both genotypically and phenotypically normal. No more issues about cystic fibrosis for this person or his or her descendants. There will be two individuals who are heterozygous, and these individuals are carriers. They don't have the disease but they do have a mutant allele. So depending on whom they marry, I shouldn't generalize, depending on whom they have children with they may or may not have to worry about the fact that they have a mutant CF allele. And one, on average, out of four will have two disease alleles and develop CF. OK? So we're going to now think about these diseases in inheritance looking at pedigrees, which I think are probably familiar to most of you. They're described in your book as well. But I just want to make sure you understand what the symbols mean before we get into it. Females are represented as circles. Males are represented as squares. Seems appropriate. Lines between them represent a mating, they have mated. And the offspring are represented below. If the symbol is left open then the individual is normal, genotypically and phenotypically. If the individual has a shaded symbol they are affected, they show disease symptoms. And if the symbol is partially shaded, I usually fill in half the symbol, the book will sometimes put a little circle inside the circle then they are carriers. They're heterozygous carriers. OK? So let's look and think about this example up here. We talked about two individuals who were both heterozygous for the disease allele, so they were carriers. Their genotype was CFD, CF wild type. They mated. They had four children. There were four possible genotypes, rather three possible genotypes. And, in fact, we observe all three genotypes in this generation. We have one individual over here who doesn't have her symbol filled. We have another whose symbol is fully filled. And then we have two whose symbols are partially filled. What's the genotype of this individual? Wild type, wild type. This one? D, D. This one? D, wild type. And this one? Same thing. OK? So that's what pedigrees look like. Let me show you a larger pedigree and give you some of the rules that apply to autosomal recessive diseases. So here's a large pedigree involving an autosomal recessive gene, disease gene. Again, the two parents are heterozygous. They had many, many offspring all here. Roughly a quarter of them will carry both copies of the disease gene and develop disease. Among the rest there will be unaffected individuals but of two classes, ones that don't have the disease gene at all and ones who are heterozygous carriers. Two-thirds of those unaffected offspring are themselves carriers, two-thirds. Two-thirds of the unaffected offspring are themselves carriers. Among those half of the offspring of a carrier are themselves carriers, as shown here. So if this individual came to you at a genetics clinic and wanted to know what is my likelihood of carrying the D mutant allele, you'd be able to say, without knowing the genotype of his mother you would be able to say that it's half of a half or a quarter. OK? Now, every once in a while two affected individuals, two homozygotes have children, as shown here. And you can see in that scenario all of the offspring have disease because the only alleles that are present are the mutant alleles. That has to be true because these are both affected, and therefore all of the offspring will also only inherit mutant alleles and develop the disease themselves. OK? And importantly because the disease gene is coming in on chromosomes 1 to 22, one of those and not the X or the Y, there is no gender associations here. Mothers can pass the disease to their daughters and their sons. Fathers can pass the disease to their daughters and their sons. There are no gender associations with this scenario. OK. Disease alleles are present in different frequencies in the population. Some of them are extremely rare, but some of them are actually rather common. So we're talking about the allele frequency, the percentage of alleles among all of our chromosomes that are the disease type. For PKU it depends a lot on where you're from. For example, in Turkey it's actually rather common, one in 206,000 alleles of this gene are mutant in this population, but in Japan it's much less common. One in 220,000 Japanese carry this allele. Exactly why this is we're not entirely sure, although I'll give you some speculation in a little while about what controls the frequency of these actually rather deleterious alleles. CF is fairly frequent. One in 25 alleles are CF. One in 25. There are as maybe 200 of you in this room, so that's some number of mutant alleles among you. It's a fairly high number. You're sitting there carrying a mutant copy of the CF allele. This is actually relevant to European descendancy. I'm not entirely sure whether it applies to all descendencies but let's just say that it's roughly that number. And another disease that you might have heard of Tay-Sachs disease in the Ashkenazi Jewish population -- -- is also quite common. One in 25. It's so common, in fact, that in certain parts of the world individuals undergo genetic testing before they decide whom to date because they want to avoid the risk that they're going to date somebody else who carries such a mutation. This is actually a very horrible disease. I shouldn't joke about it. So they want to avoid ever having to face the problem of having a child who has Tay-Sachs disease. So they undergo, in a sense, pre-marriage counseling to figure out their genotype to decide whether or not to date. And you can also imagine with similar tests we could figure out whether or not individuals carry mutations in these genes to let them know what their risks of developing disease are or whether or not to maintain a pregnancy of a child who may or may not be affected and so on. So they're very serious societal implications for these kinds of disease alleles. Now, some of the alleles are very rare. Here's one example in the Japanese population of PKU. The likelihood that two individuals would randomly get together who had mutant alleles of a phenylalanine hydroxylase gene and have a child is exceedingly small. And so in these situations, where the allele frequency is extremely rare, when you find an affected individual it's almost always a sure sign of inbreeding. Consanguinity is the term used in genetics where cousins or other relatives marry and have children. And since they are related and have a higher allele frequency within their families of a particular allele then the likelihood that they'll have an offspring who has two copies of the allele is much higher. And so when you see a pattern such as this, it's a fairly clear indication that you're dealing with an autosomal recessive disease involving consanguineous mating. Here are two cousins who are producing offspring both of whom are affected. And when you see a pattern like this you can actually figure out, or at least figure out pretty well who were the carriers in this scenario, who were the carriers in this family. Since both, since the children have two mutant copies then both of their parents must be heterozygous carriers. OK? That goes without saying. They're heterozygous. Since this individual is heterozygous and the allele was passed on from their grandparents then his mother must also carry the allele inherited from one of the two grandparents. Likewise this woman's father must carry the mutant allele. And in this generation we actually don't know whether it's the male or the female who carries the mutation. It could be either. And it's been passed along to both sides of this family tree and reduced to homozygocity in this generation. OK? You can also begin to figure out what the likelihood of other members of the family tree is being heterozygous. So this individual here has a one in two chance. One of these two parents is definitely a heterozygote, and so the likelihood that he's a heterozygote is one in two. And among his children you could say they have a one in four chance. If he has a one in two chance then there's a one in two chance that he'll pass it onto them, so overall there's a one in four chance that they will be themselves carriers. And this, again, this kind of genetic testing is done frequently to figure out what your relative risk of developing a particular disease are. Now, as I said, for other disease alleles like CF and Tay-Sachs, the allele frequency is actually strikingly high. And yet if you're homozygous for these mutations you're dead. Not necessarily right away but not for very long. It clearly reduces your reproductive fitness. And so why would these alleles be present in our population at all? Why wouldn't they be removed through natural selection? We're not going to get into this in great detail, but there are theories out there and some evidence to support them that there might be actually an advantage in certain circumstances for being heterozygous wild type over mutant. And there are theories both related to Tay-Sachs disease, sickle cell disease and cystic fibrosis such that if you are heterozygous you actually survive better in the face of certain pathogenic exposure than do people who are wild type for both alleles. And that's the argument for why these alleles actually built up, at least in the past, over the evolution of our species. OK. Let's transition now to autosomal dominant diseases. This is a famous example, Huntington's disease, otherwise called Huntington's chorea. Relatively rare. About one in 10, 00 to 25,000 individuals affected. It exhibits an autosomal dominant pattern of inheritance, as you'll see in a moment. The age of onset is about 35 to 40 years of age. These individuals actually are totally normal for the first three to four decades. You wouldn't know that they had a disease. But starting at that time they begin to develop symptoms which involve both effects on their personalities but more importantly effects on their movements. That's what you first begin to see. That's what chorea means. It's sort of this dance-like movement. And then that gets much, much more severe and stereotypical. And eventually, in addition to that, there's death of cells in the brain. And the combination of these affects leads to the death of the individual by about the fourth or fifth decade of life. This is actually a movie of an individual who has Huntington's disease. He's being told to hold his arms straight out, but because of this neurogenerative process that's taking place within his brain and also other parts of his nervous system he's unable to do so. And this is sort of the stereotypical presentation of Huntington's chorea. They have this wave-like movement and also their limbs get stuck in particular postures, as you can see this individual here. At the molecular level the problem in these individuals is that their brain cells are dying, particular ones, actually, within a particular region of the brain surrounding this ventricle here. And this is a normal space in a normal brain. And there are various sets of neurons on either side of those ventricles. And in HD patients those cells are progressively lost over time, and when those cells are lost you lose motor control and you also develop rather severe dementia. And the reason that those cells are being lost is that the mutant protein, the mutant form of this protein called Huntington, the mutant form builds up inside of those cells and aggregates and causes the cells to die. OK? And this is why this is an autosomal dominant disease. If you have the disease allele then you will get the aggregation of the protein and you will develop the disease. So let's take another example of a cross between an individual who is normal, two normal copies of HD and an individual who has a disease allele and a wild type allele who is heterozygous. Is this individual diseased? He either is already or he will be in the case of HD. It's an autosomal dominant disease. If you have the disease allele you will develop disease. If we look at a Punnett Square, this individual will always transmit the wild type allele, this individual will transmit the mutant allele half the time the wild type allele of the other. These individuals will be wild type D, wild type D, wild type, wild type, wild type, wild type. So you'll get half diseased, half normal. OK? A rather different picture than we saw previously. And importantly, as I mentioned, the presence, the mere presence of the D allele leads to the production of this toxic protein. And ultimately brain damage. And that's why it's dominant. OK. So let's look at a pedigree of an autosomal dominant disorder. Here's an affected individual, a female who marries, who has children with an unaffected male. They have four children. On average half of them will inherit the defective allele and therefore develop disease. Half of the offspring of an affected parent will be affected. Importantly, the unaffected offspring of an affected parent have unaffected offspring. If you are normal, you do not inherit the disease allele, you're scot-free. Your children will no longer have to worry about this disease. But in this case, in this individual, again roughly half, in this example two-thirds of the offspring do develop the disease. This is another really important case of genetic testing. This guy might have wanted to know that he was going to develop Huntington's disease in order to decide whether to have children in the first place. This individual here likewise might want to know before the disease actually manifested itself what would happen in order to make lifestyle decisions. Am I going to quit work and have a good time for the next ten years? Because pretty soon I'm actually not going to be able to. So genetic testing actually can make an extremely important set of decisions for individuals affected in this way. And there's actually a subtlety here, too. Sometimes people don't want to know because there's actually nothing to be done for them. In the case of Huntington's disease that's true. We don't have a cure for it. So Arlo Guthrie who is the son of Woody Guthrie, who died of Huntington's disease, apparently doesn't want to know because if he learns that he's going to get it he's just going to be depressed. If he learns that he didn't get it he might be relieved, but he doesn't want to take that chance so he's just leading life in hopes that he doesn't have the disease allele. Now, I actually don't know in his case whether he has children or not. He has children, so he actually made that decision almost for his children as well. So important implications for these kinds of genetic diseases. Now, here's an interesting pattern that I want to share with you. And this relates to a phenomenon called penetrance. Penetrance. Penetrance is a number which reflects the percentage of individuals who have the disease genotype who end up getting the disease. I've been telling you about examples where that's 100%. If you have the disease genotype you get the disease. But that's not always true. Sometimes you can have the disease genotype, but because of other factors like environmental factors, what you eat, what you get exposed to you actually don't develop the disease. Or maybe you just got lucky because some of these diseases are stochastic in nature. And you might have been one of the lucky ones. That would be an example of lack of penetrance. You have the disease genotype but you don't have the disease itself. And this can lead to the development of individuals in pedigrees, such as the one I'm going to show you, who are obligate carriers, obligate carriers. We call them obligate carriers because they have a child who is affected but they themselves were not affected. And they have a parent who likewise was affected. So the simplest explanation for a pedigree such as this is that this mother passed along the disease allele to her daughter but she did not manifest the disease, an example of lack of penetrance, but she still had the disease allele which she passed onto her daughter who developed disease. OK? And we call these individuals obligate carriers. Even though they don't manifest the disease they must be carriers. And in that sense this circle should be shaded. We haven't shaded it because actually there are other explanations for how you can get this pattern. Sometimes, for example, the disease is such that there are non-familial forms of the disease which complicate the analysis. Heart disease is a good example. There are familial forms of heart disease and there is sporadic heart disease. Maybe this woman doesn't have the predisposing mutation and her daughter just developed heart disease. That can happen. Another example is that maybe she picked up a new mutation. Her mother is actually clean but she picked up her own mutation in the development of the sperm or egg that gave rise to her, and then she has the disease. That would be rare but not unprecedented. And the final example, which is the most interesting, is so-called non-paternity or even non-maternity. So we're making an assumption here based on what the family has provided us in terms of this pedigree that this girl is the daughter of this mating, this woman and this man. But about 10% of kids born in this country actually have a father who isn't their father. It's a shocking statistic, I know, but it's true. This is called non-paternity. So it might be the case that this woman actually doesn't have the mutation. It's just that her father, her mate, the father of this girl [LAUGHTER] is not that guy. And this is sick but true, most often in situations like this it's Uncle Bob or brother Steve. I know it's disgusting but this is often an example, the explanation for examples such as this. And another interesting example is non-maternity. We're assuming that this is the mother of this child but sometimes families don't want to admit, for example, that this girl had a baby. And so they give it to Aunt Sue. And now Aunt Sue's child gets the disease, but it's not because of Aunt Sue's genes. It's because of her cousin. OK? So here are some examples. Now, I have one more minute, and I need to riffle through the slides because I'm going to leave you for a few days. And I want to just remind you that there are X linked diseases that affect, that are both dominant and recessive. Most of them are recessive. And here's a classic example, an X linked recessive disease involving hemophilia in the royal families of Europe. Importantly, when you look at X linked disease pedigrees, and here's Duchenne muscular dystrophy, another familiar disease, again X linked recessive, there are certain rules that dictate the development of the disease over the generations. They're summarized here. And you should look in your book for more information. And, finally, there are rare examples of X linked dominant diseases, and their rules of inheritance are somewhat different. So you should familiarize yourself with X linked modes of inheritance.