Flash and JavaScript are required for this feature.
Download the track from iTunes U or the Internet Archive.
Lecturer: Prof. Tyler Jacks
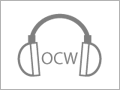
5: Biochemistry 3
Related Resources
Lecture 4: Biochemistry 2
Lecturer: Prof. Hazel Sive
No audio track available
OK. So we're going to continue with the discussion about biochemistry, and specifically focus on enzymes today. Professor Sive introduced those to you briefly in her last lecture. I'm actually covering for her today. This is one of her lectures but she has given me her material, so hopefully it will go fine. She wanted me to remind you a little bit about energetics, specifically that a negative Delta G in a reaction implies that the reaction can occur spontaneously, that is if the products have lower energy than the reactants. And so given enough time this will happen in that direction. But importantly for many reactions an activation energy might be necessary to get that reaction started. And if the activation energy can be sufficiently high then in a reasonable amount of time the reaction will actually never proceed forward. And it's the job of enzymes actually to deal with that problem, as we'll discuss. So this is the third of three lectures in biochemistry. We're going to transition next week to genetics, and I'll be actually teaching that section on genetics. Before we get into today's lecture proper, Professor Sive wanted to quiz your knowledge from previous lectures. So firstly in this structure, this polypeptide structure, the question is was the valine amino acid, the Val, added last or first in this reaction? Specifically true or false, was it the last amino acid added? False. Very good. It was the first. Because amino acids are added in polypeptides from the amino end to the carboxy end. And in this case Val is next to the amino ends. So the second question is in this reaction shown here, the generation of sucrose from glucose and fructose, which has a positive Delta G, does this release energy or consume energy? Specifically true or false, does this? False. Good. So the Delta G is positive, which means to get this reaction to go you need to add energy. And there are various ways of adding energy. In a synthetic case a chemistry example you might add heat or you might link this to another reaction that actually produced energy. And finally is activation energy required only to initiate reactions with a positive Delta G? No. Very good. So, as we just talked about, activation energies can be important both for reactions that consume energy and give off energy. And so even in situations where reactions give off energy, it may be necessary for an enzyme to deal with this activation energy problem in order to speed up the rate of the reaction. OK. So Professor Sive gave you examples previously comparing the cell to a factory. And I think it's a very apt analogy. And it's particularly apt with respect to the subject of today's lecture which are enzymes. There's a great deal of interest these days in the MIT community and around the research community around the world in nano technology, building tiny little machines that can do work. Well, I would say that proteins are the consummate example of nano technology. These are small entities, five to ten nanometers in diameter, which carry out very specific and very powerful reactions. So proteins are already nano particles to the nth degrees. And the cell is itself remarkable in this respect because the cell, which is only ten to thirty microns in diameter not only has all these nano particles doing all this work, but it also contains the blueprint information for building those. It has the synthetic capability for making the raw materials that they act on. And it has the micro machining capability to build the nano machines. And this all takes place in a very, very tiny space. So the cell is an unbelievable example of engineering that we can only just aspire to replicate. OK. So, again, the subject of today's lecture is enzymes as biological catalysts. And I want to introduce this by putting some of the earlier stuff into perspective. So Professor Sive told you in her last lectures about macromolecules -- I'm not going to talk about all of them that she's told you about, but she's talked about DNA, deoxyribonucleic acid which is responsible for information storage. She also told you about RNA, ribonucleic acid, a related polymer, nucleic acid. RNA actually has many functions in the cell. Information transfer is an important one, but it's not limited to that. RNAs can also function structurally. When we talk about protein synthesis, for example, you'll see that RNA molecules surface scaffolds for the ribosome which is the machine that makes proteins. And they can also be catalysts. This was not appreciated for a long time. We now know that in biology, in biological systems today RNAs can function as catalysts. And we actually believe, in an evolutionary sense, that RNAs were the first catalysts. Before there were polypeptides and proteins, life was made possible through the catalytic properties of RNA molecules. And your book actually talks about those in the chapter that you just read. They're called ribozymes, for RNA ribose-based enzymes. And then there were proteins. And we'll talk about proteins as catalysts today, but importantly proteins also serve structural roles. They compose, for example, the long cables that extend throughout your cells to give them the proper shape and allow them to move. They're important carrier proteins. For example, hemoglobin which carries oxygen to different parts of your body. There are proteins that bind to iron, for example, and deliver it from your diet to the relevant parts of your body that are protein specific to that function. And then there are catalysts. And it's the catalysts that we'll focus on today. Now, the way that proteins carry out these diverse functions is that by virtue of their amino acid sequence, their primary sequence of amino acids, they fold up into a particular shape. Proteins have very specific three-dimensional shapes. And these shapes then dictate their function. Depending on what the protein is supposed to do, it will have a different shape. So, for example, there are proteins that, as I mentioned, function as structural elements within the cell like long cables. They form polymers. And these proteins have shapes a little bit like Lego blocks that link together, one to the next, and as such line up into these long polymers. So some proteins have structures that just allow them to bind to one another and form long polymers. The proteins that we'll talk about today, catalysts, usually have, maybe always have a specific shape. And I'm taking a three-dimensional structure here and simply cutting a slice through it, so you're looking at sort of the middle of the protein cut in half. Here's the overall three-dimensional structure of the protein. And in this portion here, which we call the active site, is where the chemical reaction takes place. Now, we know a lot about many enzymes, as well as other proteins in the cell, based on the primary amino acid sequence that we can determine from the genes sequence. But we also have seen what these look like using methods in x-ray crystallography. We won't actually review the methods of x-ray crystallography with you, but basically sufficed to say that you can take a pure protein, allow it to form into a crystal, shine x-rays through it, the x-rays get diffracted based on the position of the atoms in the protein. And you can then interpret that diffraction pattern to tell you what the structure of the protein was. So these are not just cartoons. We actually know what these proteins look like in great detail. Not only do we know what the proteins look like in many instances, but we also often know what the protein looks like in complex with some reactant to which its bound. And these we call co-crystals. Crystals that occur between the protein and the substrate molecule that it's going to act upon. And so we have a very clear idea of what the chemistry is that's going on inside the enzyme's active site. And this information is not just useful for biological purposes, but the more we understand enzymes and the specific structure of enzymes the more we can do about it. So in disease, for example, where you have an enzyme that's causing some pathogenic problem, if you want to inhibit that enzyme, the more you know about what's happening here the more precise you can be in your design of an inhibitor. And that's now happening, and I'll tell you an example of that later in the lecture. Now, I'm just curious to know whether you have a sense for why the protein has its particular structure, and also what distinguishes one active site from another? There are thousands of enzymes inside this cell. Do they all look like this or does each one look different? Are they all the same? No. They're all different. They're different in shape, as I indicated earlier because their primary amino acid sequence causes them to fold up based on local interactions between amino acids into helices and beta pleated sheets. As I told you, they interact with each other. And that gives them that large sort of three-dimensional structure. But it's the nature of the amino acids in this active site which ones are there. Is it a glutamic acid, a valine, a methionine? Which specific ones are present there and how are they oriented which allows them to bind to particular molecules, substrates, and do chemistry on them? And the reason that proteins are much better catalysts, or much more powerful catalysts than RNAs, is that our RNAs are fairly boring. They only have four subunits. They don't have that great diversity of chemical reactive groups that you find in proteins. Proteins, as you heard, are composed of 20 distinct amino acid subunits. They are all differently chemically in those R groups that Professor Sive told you about before, and they can do different chemistry in the active site. So different enzymes are different by virtue of their overall structure and the particulars within the active site that allows them to do what they do. I wanted to mention briefly that we often use the suffix ìaseî to designate an enzyme, polymerase, DNA polymerase, sucrase. You'll see those terms all the time. Whenever you see it, it reflects that the protein is an enzyme, the suffix A-S-E. OK. Again, just for perspective, where do the proteins come from in a sense? How does the cell know what to make? We're going to get into that in later lectures, but just so you have a sense of it, the information to produce a particular protein with a particular amino acid sequence, and therefore shape and therefore function, is encoded in the genes, which are in the DNA. This information is transferred into an intermediary molecule, which is RNA. Again, you're going to learn about these details. You don't have to worry about them so much right now. You'll learn about these details later in the class. And the particular RNA molecule that carries the information from the DNA is called the mRNA, messenger RNA. And that mRNA is then translated into the protein itself. So the reason that we have proteins of particular sequence and particular shape and particular function is that we have different genes that carry the information to make those specific proteins. OK? And we'll see that again in detail in later lectures. OK. So very importantly we talked last time, you talked last time about the energetics of reactions, as illustrated here, that in many reactions there is the energy of the reactants themselves, the energy of the products, as well as so-called activation energy. That is the energy that's required to make that reaction go, which can be greater than the energy of the reactants. The important function of enzymes is to lower the activation energy to reduce the threshold that these reactants have to go over in order to carry out the reactions that lead to the products. The enzyme's function is to lower the activation energy. And the way that enzymes do that is several-fold, as we'll review. The most important thing I can imagine you can take away from this lecture is the understanding that what enzymes do as catalysts is to lower the activation energy. They don't change the nature of the reactants, they don't change the nature of the products, they actually don't change themselves in the course of the reaction, but what they do is to facilitate the reaction by lowering the activation energy. And that is the nature of catalysis. So enzymes are biological catalysts. Their function is to increase the rate of a reaction. As I said, reactions that release energy will happen spontaneously, but it might take a very long time. Enzymes function to increase the rate at which those reactions can happen. And they can do so in impressive ways. They can increase the rate by a million-fold. So they really can change whether a reaction will take place in the lifetime of an individual compared to whether it would take place in microseconds. OK? And many biological processes have to happen within the course of microseconds or seconds. And, therefore, without enzymes those would not be possible. Importantly, as illustrated on this slide, enzymes do not change the Delta G. They don't change the Delta G of the reaction. Delta G is the same. What's being changed here, in the presence of an enzyme, a catalyzed reaction, is the activation state. The energetics of the products and the energetics of the reactants don't change, so the Delta G does not change. So, again, they do so by lowering the activation energy. And this is often done by combining the reactants with portions of the protein to create what's called a transition state complex. And it's the nature of that transition state complex, the protein bound to the substrates that allows the activation energy to be reduced, as you'll see in a moment. I want to emphasize that the enzyme itself is the same at the end of the reaction as at the beginning. The enzyme does not change. It goes through one reaction cycle. It's exactly as it was when it started. And that's important because it means that the enzyme can be reused. This is not a single reaction process. The enzyme can be used over and over and over again, which is another part of the definition of a catalyst. OK. When we talk about enzymes, reactions, reactants and products, we use slightly different nomenclature, and so it's important that you see that and get to recognize it. The enzyme, often denoted as E, combines with substrates, one or more substrates, described as S, to form a complex which is designated ES. That's where this transition state is taking place. And then following that the enzyme releases the products. And, importantly, the enzyme can then be recycled to do this process again on new substrates to produce new products. So the S in this is the reactant or substrate, and P is the product, and the ES is the enzyme transition state complex. As I said at the beginning, enzymes have very particular specificities. They are designed to do specific reactions. They don't bind to every old molecule in the cell. And this is determined, as I said, by the shape of the enzyme and its complementarity to the substrates to which it binds. And that's illustrated here on a slide from your book where you can see an enzyme with its active site. And here are three potential substrates. Based on the shape of the active site and the particular side chains on those amino acids, the yellow one and the red one will fit into the active site, but the green one will not. So specificity, in this case, is determined by the complementarity between the shape of the substrates and the shape of the active site. And then, by virtue of their positioning within the active site, the enzyme is now catalyzing the binding, covalent attachment of the yellow one to the red one to produce the product, as shown here. So specificity is achieved by the complementarity between the active site and the substrates. The transition state is achieved by a variety of conditions that the enzyme places upon the substrates. So the substrate fits in much the way a key fits into a lock into the active site. This then promotes the formation of this transition state. And that is done by three distinct, sometimes related but, distinct mechanisms. One is the fixing of the orientation of the two substrates to one another. They're not just randomly floating around in solution anymore. They're literally aligned next to each other in a way that will promote the chemical reaction. And that's one way that the activation state is lowered because now you don't have the problem of kinetic energy of the molecules floating around. A second is what's referred to as induced fit, and in your book referred to as strain. And I'll show you a slide of this in a second. And this is important because often times the activation energy is due to the fact that the molecules have to get contorted. It's not a native confirmation of the molecules during the chemical reaction. They actually have to get bent in ways that they don't like to be bent. The enzyme helps this by adding chemical groups around it which promote the bending process, promote the sort of straining of chemical bonds that allows additional reactions to take place. So the enzyme produced is this so-called induced fit. And, finally, the enzyme can, depending on the nature of the chemistry, apply charge. We know that there are charged amino acids, both positively and negatively charged amino acids. There are acid-base reactions that often take place within the enzyme's active site. So the presence of charges can facilitate those acid-base reactions. They can donate positive charge or donate negative charge to allow the reaction to take place more rapidly than it would do spontaneously. And those are illustrated in a following slide, actually. This just shows you an example, a specific example of a reaction that is catalyzed by a particular enzyme. Here we have the substrate. It happens to be a sugar, a disaccharide sugar, sucrose, and is made up of the subunits glucose and fructose for you to use sucrose, which you can eat. To produce energy you need to break it down into glucose and fructose. And this reaction is catalyzed by a particular enzyme called sucrase. And you can see in diagrammatic form what happens here. Here's sucrase. Here is its active site. You can see that its structure is exactly complimentary to the substrate. So the substrate now floats in, binds to this active site. There's then a chemical reaction, which is basically the addition of water to break this bond, which is catalyzed by the enzyme. And then the products are released, the enzyme remains as it was at the beginning of the reaction, and it can go through the reaction cycle one more time. This is the picture that shows you the various ways that the active site can promote the transition state complex. One, as I mentioned, is orientation. Again, two substrates here are positioned next to each other in the way that we want them to react. So that's helpful. A second is the straining process, the fact that the protein can impose, in a sense, stress on the molecules, the substrates, change their shape, and in that way facilitate the chemical reaction, and finally, as I mentioned, to charge. In the case of acid-base reactions there are positively and also negatively charged amino acid side chains that can contribute their positive or negative charge to facilitate the reaction. And this is one final example. Here we're talking about an enzyme that adds a phosphate group onto glucose in an early step in glucose metabolism. And, in this case, the enzyme actually changes its shape as a consequence of the substrate binding to it. This happens [UNINTELLIGIBLE PHRASE]. At least he didn't point to me. [LAUGHTER] What was that all about? I was actually prepared to have those sorts of interruptions on Monday which we always have. I don't know what that was all about. So what was it all about, pretty boy? [LAUGHTER] And who is pretty boy anyway? Somebody back there. OK. Well, that was fun. Anyway. Well, we were talking about enzymes. So, again, some enzymes, as indicated here in the example of hexokinase, will actually change their shape in response to the binding of the substrate, here glucose. And this is maybe a more interesting example of something I'm going to come to later, which is that proteins are not static in their shape. They actually do change a little bit, and the ability of them to change can tweak, can tune their activities so that positioning the exact structure of the active site can change based on other things that are happening in the protein. And that's a useful thing with respect to regulation. You can turn up the activity of an enzyme. You can turn down the activity of an enzyme based on the changes that the overall structure can make. OK. And then just to bring the subject of specificity of enzyme function home, here is an example from real life. This is a packet of Equal, which you may use. It's an artificial sweetener. And you may have noticed on the very back of the Equal packet it says phenylketonurics: contains phenylalanine. And you might have wondered, what the hell is that all about? Does anybody know? Is anybody a phenylketinuric? You don't actually have to say if you are or not, but does anybody know what this means? Yes. It's close. You're actually thinking of a similar disease called alkaptonuria. But you're on the right track. Right. You cannot break it down. And specifically what you cannot break down is phenylalanine. This is a disease that affects only about one in 12, 00 individuals. It's a so-called metabolic disease. We'll talk about metabolic diseases later. And the important point here is that the enzyme that's responsible for breaking down phenylalanine is altered in these individuals in one residue, one amino acid out of 451. Protein has 451 amino acids. One of those amino acids in the active site is not what it's supposed to be. And therefore it cannot bind properly to phenylalanine. And that causes a defect in the breakdown of this and the build up of a toxic substance, as I'll show you in a second. This is NutraSweet. You might not have known that it is a dipeptide composed of aspartic acid and a phenylalanine linked together with an extra group on it, probably to make it more soluble or ability to pass through cells more easily. In your body, when you take in phenylalanine from the diet like with respect to NutraSweet, when NutraSweet gets into your body, the phenylalanine and the aspartic acid get broken apart so you have increased phenylalanine, but however you intake phenylalanine from your diet it's normally converted enzymaticly by an enzyme called phenylalanine hydroxylase which converts it from phenylalanine to tyrosine. And the tyrosine is either used for stuff or it's broken down itself. It's actually used for making melanin. And so these same patients who I have just mentioned, these phenylketonurics also have lighter hair and lighter skin because they cannot make as much tyrosine, and therefore don't make as much melanin. But the real problem is not that. The real problem is that if you have too much phenylalanine in your blood because you cannot break it down properly. Then you build up phenylpyruvic acid which is a natural byproduct of phenylalanine. And this stuff is toxic when present in high levels. And so the patients, these phenylalanine hydroxylase mutants, which are now called phenylketonurics, have a defect in this enzyme, cannot carry out this reaction properly. And therefore this spontaneous reaction happens more readily. And therefore you have high levels of this toxic compound that causes mental retardation, actually. It causes some sort of neuro toxicity. And that's how it was originally defined. And the reason we're telling you about this is that this is an example of enzyme specificity because this enzyme is different, as I said, in only one of 451 amino acids. The 408 amino acid is supposed to be an arginine, and instead is a tryptophan. And as a tryptophan it cannot properly bind to or carry out the chemical reaction. And therefore the enzyme fails, levels build up, and the individuals have a very, very severe phenotype. A very, very severe disease presentation, I should say. Now, I indicated a little bit ago that enzymes can be tweaked in their function. Enzymes are not just static in their ability to interact with substrates and catalyze reactions. In fact, they are highly regulated. And they're regulated by a number of different both external and internal processes. So regulation of enzyme function is critical with respect to producing the right sort of products at the right sort of times and rates within your cells. And one key factor that determines the regulation of a given enzyme is the pH, the pH of the solution that the enzyme finds itself. Now, why would that be? Does anybody have a sense for why that would be? Why does the pH of the cell's environment determine the enzyme's function? Yeah. Say it again. Right. So proteins can denature at, extreme pHs in both directions, actually. But more importantly they're optimized based on the side chains that are present within the active sites. So, as I said, there are charged amino acids which have different pKas. And depending on the pH of the solution, they'll either be protonated or not protonated. And their state of protonation, whether or not that they have a proton bound to them, will affect their ability to carry out the chemistry. So enzymes are perfected to function in the pH conditions they find themselves. So, for example, salivary amylase, which breaks down carbohydrates in your saliva, has a pH of around seven because your saliva is around pH seven. So it's been evolved to function best at that pH. If you increase the pH or decrease the pH it doesn't work so well, as indicated by this reduced reaction rate at higher and lower pHs. In contrast pepsin, which is an enzyme that is present in your stomach acid and in your small intestine where the pH is very, very low, works best at low pH. If you raise this to increase pH, it doesn't work at all well because presumably at the increased pH things that should be protonated are not protonated. And now those reactions that should be taking place in the active site don't. This is also another interesting example of regulation in the sense that pepsin breaks down proteins. And you actually don't want rampant protein breaking down enzymes floating around in your body. Particularly, you don't want them in the cells that make pepsin. You could imagine that the cells that make pepsin run the risk that pepsin is going to eat all the proteins in those cells. It doesn't happen because the pH of those cells is around seven, and therefore the pepsin isn't active. It only becomes active when it gets dumped into the acid environment of the stomach and the small intestine. So that's another justification for tweaking the activity of enzymes. Another example is temperature. Again, based on the structure of the protein, which is strongly influenced by the temperature, proteins have optima. Most of our proteins function best at what temperature? 37 degrees Centigrade. 98.6, or whatever, Fahrenheit. But that's not true of all organisms. As I mentioned in the first lecture, there are organisms that live in thermal vents where the regular temperature is 80 degrees centigrade or higher. Their enzymes actually work like crap at 37 degrees, but they work great at 72 or 75 degrees. They've been optimized, based on their structure, to function best at the temperature in which they find themselves. And finally, or almost finally, covalent modification. And there are different ways that proteins can be modified after they've been made in the translation process. Other stuff can get added to them covalently. And I'll give you an example of phosphorylation -- -- because it's going to come up in later lectures, too. Phosphorylation which means that the protein gets an extra phosphate group added to it. And if you imagine, for example, an enzyme, it has an active site here -- -- which is blocked at its front door. Stuff cannot get into it because this little arm is kind of hanging over the front of the active site. What can happen is that a reaction, another chemical reaction that adds a phosphate group -- Phosphorylation, which is another enzymatic reaction, can cause the enzyme to open up and allow substrates to come through. And this is a reversible process. Other enzymes called phosphatases can come along, clip the phosphate off and return the enzyme to its inactive state. And then, finally, there are partners, other molecules that the enzyme binds that help the enzyme do its thing. And these are summarized on this slide, which comes from your book. There are three groups that are shown here. You should probably be familiar with what these groups are and examples from within them. For example, cofactors. These are usually small metal molecules, atoms. Iron, copper and zinc are three that are shown here. These participate in the chemistry. They actually participate in the catalysis for enzymes that require them. And, actually, many enzymes in your bodies do require such cofactors. And that's one of the reasons it's encouraged, you're encouraged to eat zinc and stuff like that, because many of your enzymes need it. Another class called coenzymes, this happens to be a horrible name in my opinion, a really, really bad name. It's one of these historical names that we're stuck with. But these are, again, partner molecules. They're really substrates. They're really substrates in the reaction, but they're called coenzymes because they're used by many different enzymes in coupled reactions. And an example here is NAD which is involved in a hydrogen donating and receiving. And this I mention specifically because it's the product, or it's the byproduct of one of the vitamins you eat, vitamin B. And many of these coenzymes are the products of vitamins. And so that's one of the reasons it's important to eat your vitamins. And finally what are called prosthetic groups, the same word as artificial arms and legs. Prosthetic groups like heme, which is present in hemoglobin, flavins, other things, which are involved, again, in helping the enzyme or the protein do its thing. And the distinction between these and these is that they are larger. They're actually synthesized by the body, as opposed to these which are just taken up in the diet. OK. This is an example, and I'll go through it quickly for lack of time. This is an example of one chemical reaction. What we're talking about here is an enzyme called dihydrofolate reductase. This is an important enzyme in producing molecules that are required to build nucleotides in your body, both DNA and RNA precursors. Without this enzyme you cannot make those things, you would be dead. It's a very critical enzyme both in biology and in medicine. Dihydrofolate reductase happens to be one of the targets of an important chemotherapeutic agent called Methotrexate. It's used because cancer cells grow a lot. You want to inhibit their ability to grow, so you inhibit their ability to carry out this reaction to produce this relevant product. And also in bacteria it's the target for a particular antibiotic called Trimethoprim. Folic acid you know that you take in from your diet. And it's then broken down in a way, or not broken down. It's modified in a way that it becomes useful for these synthetic reactions. And that's the job of dihydrofolate reductase. Dihydrofolate has two hydrogens positioned at the seven and eight positions. And tetrahydrofolate has four hydrogens at these four positions. And it's the tetrahydrofolate that you want to use, that you need to use for these subsequent reactions. So the enzyme then, dihydrofolate reductase adds hydrogens. It reduces dihydrofolate to tetrahydrofolate. And it utilizes a cofactor NADP shown in green here to do that. It's the NADP, NADPH which transfers the hydrogens to the dihydrofolate. This is a little bit hard to see because it goes pretty quickly. You might look at it on the Web or at home. This is the dihydrofolate coming in. You can see in white the enzyme actually moving. It moves in order to carry out the chemical reaction. And you can see in green the NADP, initially NADPH coming in, interacting with the dihydrofolate, transferring the hydrogens and allowing it to become tetrahydrofolate. OK? So that's one example of an enzymatic reaction. OK. In the final eight minutes, and we do have a lot to get through, so if you could just hang in there until five of that would be good, I want to talk about another form of regulation, and that is specifically the existence of inhibitors and activators. So, again, the regulation of enzyme function is extremely important. You want to make sure that the enzyme is working at optimal rates, higher or lower, depending on the circumstances. And this can be adjusted naturally inside the cell by other molecules that can function to inhibit the enzyme or to activate the enzyme. And, as I said, we can also do that medically by making inhibitors or activators that change the activity of enzymes inside our cells. Terminology. These inhibitors and activators can be reversible or irreversible. They can either bind and come off and bind and never come off. This takes the enzyme out of play. It goes to the bench. It cannot work anymore. This one can come back off and the enzyme can continue to function. They can be competitive. And I'll show you what this means in a second. Competitive -- -- versus noncompetitive. And, again, I'll tell you what that means in a second. Competitive is illustrated here. If this is the active site and this is the substrate which would normally fit into that active site, a competitive inhibitor, like the name suggests, competes with the active site. It gets in there and prevents the substrate from binding. Pretty simple concept, right? A noncompetitive inhibitor functions by binding somewhere else on the protein and changing the structure of the active site. So here again is the substrate. It could bind to this active site, but when the noncompetitive inhibitor binds over here it changes the active site. And now the substrate cannot bind. So it's not competing with the substrate directly, but it's affecting the ability of the substrate to bind to the active site. Now, these noncompetitive inhibitors, and you can also have molecules that activate the enzyme at these other sites, are binding to portions of the protein that we call allosteric sites. A term you should be familiar with, allostery, allosteric regulation. And what this is, again, are molecules. Sometimes they're products of the reactions. Sometimes they are other things that bind somewhere on the protein, not at the active site, which change the nature of the active site. So in this example we see an allosteric site next to an active site. The binding of an activator can lock this enzyme, it happens to have four subunits, into a confirmation where the active site is open. Or another small molecule, an inhibitor can bind to the allosteric site and lock the protein into an inactive confirmation. OK? So allosteric regulation, sensing some other molecule and changing the activity of the enzyme. Why would you want to do that? Before I get to that, let me give you one real-life example of inhibition. It comes from my world. Cancer drugs increasingly are becoming more specific, and this is the best example. I don't have time to go into it in detail now for lack of time, but this is a drug made by a local pharmaceutical company called Novartis which binds to one of these kinases, these phosphorylating enzymes, important in a particular type of cancer. It is a competitive inhibitor of ATP. ATP needs to bind to an active site for this protein to function. The drug called Gleevec is a competitive inhibitor of ATP, therefore ATP cannot bind, therefore the enzyme cannot function, therefore the cancer cells cannot stay alive, therefore the cancer patient is cured. Great example. It happens to be true. So this is not just theory, not just bench biology. This is real-life in pharmacy in this example. And this is a three-dimensional picture of Gleevec in green bound to the active site of the kinase called able shown in the red and blue ribbons. OK. So, again, feedback regulation. The reason that we have allostery and changes in regulation relate to a diagram like this. And the important points here are that these enzymes that we've been talking about almost never function in isolation. They're almost always in pathways. Something produces product one, the next enzyme works on product one to make product two, and so on and so forth. And you actually, the cell wants to coordinate the activity of these enzymes so that the right amount of product is made at the bottom of the process. It's like the regulation of an assembly line in a factory. You don't want to make too many tires if you don't have enough cars. So when you have enough tires you feedback the tire generation and you bump up the car generation. The same thing happens in biology. This pathway A goes to B goes to C. C splits to D and F goes to G goes to E. Depending on how much G you have, you might feedback on this enzyme to make less F. And you might feed forward, you might have positive feedback on this enzyme to make more E. And this regulation is done by this sort of allosteric process whereby the G product to an allosteric reaction might inhibit the enzyme producing F and might activate the enzyme producing E. And this example shown here from an actual metabolic process, the generation of isoleucine is a specific example whereby when you make enough isoleucine the isoleucine will bind back to the enzyme way up here in the pathway to shut it down. Once I have enough isoleucine, isoleucine binds to this allosteric site on this enzyme, which now slows down the production of these intermediates, and therefore results in the production of less isoleucine. I left off two slides on energetics and ATP, but I'll mention those next time.