Flash and JavaScript are required for this feature.
Download the track from iTunes U or the Internet Archive.
Lecturer: Prof. Tyler Jacks
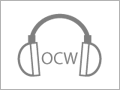
14: Recombinant DNA 2
Related Resources
Biology terms (PDF)
OK. So last time I reviewed for you some of the basic cloning techniques. I failed to show you this slide, which I intended to do, which is a commercially available plasmid. I told you about plasmids last time, small circular DNA molecules that are used in the purpose of cloning. They're derived from nature, but then they've been heavily manipulated by scientists to be useful for the purposes of cloning. Recall that they have two, three critical elements, an origin of replication to allow them to be replicated inside of bacteria, a selectable marker, a drug-resistance gene, ampicillin resistance gene for example, and finally, can you now show the middle slide? He's working on it. And finally what we call a multiple cloning site, a set of restriction sites, restriction enzyme recognition sites that allow us to pop in pieces of DNA. I have dual paper readers. Dual paper readers? That doesn't happen very often. You usually have one but not often two in a row. Good. So these are useful practical tools. Maybe before I forget, I'll mention, remind you of the quiz on Monday, where you go based on your last name. There was a discussion last time about the review session, which is actually not on here but we didn't change the review session. Oh, because it was last night. We weren't able to change the review session, which was last night, but there is a tutoring session which will cover the same material from today between 4:00 and 6:00. And then there are additional sessions with the TAs, including up till Monday morning before the exam. So there should be ample opportunity to get your questions answered. So plasmids are now sort of a useful and are a very available tool. This figure comes from your book. It's a version of what we covered in our example last time, the cloning of the T gene. Here in red is the starting material, the DNA from the source. In our case it was S. pyogenes, that flesh eating bacterium, but it could be anything. It could be genomic DNA from you. You take that genomic DNA, whatever the source material is, you digest it, cut it with a restriction enzyme, with a particular restriction enzyme. You then mix that DNA with plasmid DNA which has been cut with the same restriction enzyme, the sticky ends of the linear molecule anneal with the sticky ends of the plasmid. You then add DNA ligase to seal those nicks to produce full covalently closed circular molecules that are composed of both the plasmid and now an insert from the DNA sample. You then take those recombinant plasmids, mix them with bacteria under conditions which allow the DNA to get into those bacterial cells. This is a process called transformation. You then plate those bacteria onto auger plates that contain the antibiotic, in this case ampicillin in our example. The bacteria that didn't get a plasmid die. The bacteria that did get a plasmid survive and form colonies on the dish. And then the question I left you with was how are we going to find the colony or colonies that carry the T gene? How are we going to find the gene, the recombinant plasmid, the colony carrying the recombinant plasmid with the T gene? I also want to mention a bit of terminology, which we will come back to later. We often refer to the collection of colonies, the collection of recombinant plasmids contained within those bacteria as a library. Like a library containing books, this is a collection of different things. In this case, different recombinant plasmids which you can go back to repeatedly taking isolates from the colony. In our case we would then grow up the individual bacteria to get ample amounts of the plasmid of interest. And this term library is used in molecular biology a lot, a diverse collection of clone fragments to which you can return depending on your specific needs. So how are we going to isolate our plasmid of interest? Well, if you recall, we knew the sequence of the genome of S. pyogenes. And, therefore, we knew the sequence of the T gene. So imagine that the T gene had a particular sequence starting from the 5 prime end of A-G-G-C-T-G-G-T-G-G-G-A to the 3 prime end. So this is imbedded within the T gene. The reverse complement on the other strand reading in this direction from the 5 prime end would be T, did I say that, what's this? C-C-C-A-C-C-A-G-C-C-T 3 prime. OK? So we know a bit about the thing we're interested in. We know its sequence. And we can use this information to our advantage to isolate the plasmid that carries this fragment. So the bacteria, as shown on this slide, are present in colonies. Each colony contains a recombinant plasmid. The first step in isolating the plasmid of interest is to make a copy of the plate that carries these bacteria to replicate what's on this plate onto a piece of filter paper. We call this replica plating. And in this case we're going to do it onto a filter, a piece of paper or nylon to make a copy of the colonies and the pattern of the colonies onto something else that we can manipulate. So we make an exact copy. And this done by literally placing the piece of filter paper onto the Petri dish, as shown here. Here is the Petri dish with the colonies. You place a filter on top of those colonies. They'll stick to it. Some of the cells in those colonies will stick to the filter. You then pull the filter off and you get some of the colonies, some of the cells sticking in exactly the place where they were on the original plate. You can actually place this on another plate and allow those cells to grow directly on the piece of filter paper. So you make an exact copy of what was here onto a piece of paper. Now, what are you going to do with that? Well, we're going to use this information that we have about the sequence of the gene to isolate the colony of interest. First thing we do is to lyse the bacteria that are now present on this filter and denature the DNA. What I mean by denature the DNA is to pull it apart. It's double-stranded when it's present in the bacteria. To denature DNA is to make it single-stranded. And typically the way we do that in these applications is to place a piece of filter paper under high pH conditions, and it causes the DNA strands to melt apart. So now the plasmids, which were double-stranded in these bacterial cells, were double-stranded here have now been pulled apart into single-stranded molecules. So, on this piece of filter paper there are cells with unwound DNA, single-stranded DNA in them in this same pattern, which I won't try to reproduce here. And the question is how are we going to identify which colony carries a piece of the T gene? How are we going to do that? Does anybody have a clue? All you need to know is present up here. Yeah? So you're thinking of the example from the book in which the, in the example in the book they used a plasmid that had two selectable markers. And you were interested in those that grew under one antibiotic selection and didn't grow under a different antibiotic selection. In our case, in our plasmid, we actually didn't have two selectable markers. We only had one. So we're not going to use that strategy. We have to rely on some of the information I've given you already. Well, we know the sequence of the T gene. We know, for example, this little bit of the T gene, so we can make some that we call a probe. A probe, for this application, is an oligonucleotide, a small segment of nucleotides that we can synthesize. You can actually make any DNA sequence you want using chemical synthesis. This has now been fully automated. So you can just punch the sequence into a computer, attach it to one of these machines, the machine will go off and make a linear piece of DNA of any sequence you want. It's like a typewriter almost. You type it in, and what you get back out is a piece of DNA that is the sequence that you typed in. So we can make any DNA we want such that we could make a piece of DNA that had the sequence A-G-G-C-T-G-G-T-G-G-G-A. OK? We could make that piece of DNA. Moreover, we could modify that piece of DNA with enzymes to add a radioactive phosphate at this 5 prime end. So we could make a radio labeled probe. So now that we have that, what are we going to do? Anybody? Yes. Yes. Right. Exactly. So this is now complementary to the other strand. The DNA within these cells that have been lysed and now stuck onto this filter are also single-stranded. So this complementary strand is going to be sitting in a single stranded conformation ready to anneal, hybridize with this probe. So you apply this radioactive probe to this filter. It will bind somewhat indiscriminately at first, but it will bind very tightly to that complementaryary strand from the T gene. And remember there are probably a million bacterial cells within this colony, so there will be a million copies of the probe anneal to this colony. You do have to do a little washing to get rid of the nonspecific binding which will take place. So you wash the cells, or wash the filter, get rid of the nonspecific binding. The specific binding will withstand the wash. And then you apply a piece of x-ray film. And what you'll end up with is a faint image of the filter, some very faint signal from the colonies that don't hybridize, and a very strong signal from the colonies that did hybridize properly to the probe. OK? So now you know, based on the position on that filter, which colony it is that carries the T gene. And in this case it's that one right there. So we can go back to that master plate that we've kept, use a toothpick, pluck off that colony and grow it up into high concentrations successfully having completed our cloning project of the ever toxic T gene. And that's gone through here in this figure, if you want to look at it again. You take this filter that has the colonies. It's actually the step here where you grow the cells up on the filter itself. You then lyse the cells, denature the DNA, add the radioactive probe. It won't hybridize strongly to those colonies that don't carry the clone of interest. It will hybridize strongly to the colonies that do have the clone of interest. And then through this x-ray film method you can visualize where those colonies are and then go back and pick the colonies from the master plate. OK? So that's how it works. It's fairly straightforward and works extremely well to give you the cells and clones of interest. Woops. So that's what we call clone identification by hybridization using a radioactive probe. And I should mention, because it will come up again later, that we use these radioactive probes extensively for molecular biology. And we use non-radioactive probes, oligonucleotide synthesized in the same way that I mentioned for all sorts of molecular biology applications in this era. And you'll see examples later in the lecture. I want to briefly mention another technique known as cloning by complementation. In this instance, we're going to clone by the function of the plasmid that we've introduced into the cells. Not its sequence. By its function. And the example I'm going to give you is to clone a gene that you might know about based on the biochemistry of the organism, but you haven't identified the gene that encodes that enzyme. The example I'm going to give you is to clone an enzyme which is responsible for catalyzing the breakdown of lactose, a disaccharide. And this is accomplished by an enzyme called beta-galactosidase. And it converts glucose. It converts lactose to glucose and galactose. And this is an important enzyme in bacteria and also in you. The enzyme is encoded by the lacZ gene of bacteria. OK? And this exercise that we're going to go through is to try to isolate the lacZ gene through this method called cloning by complementation. We need to use a trick, which I'll show you on this slide. And that is that there's an artificial substrate for this enzyme which is called in the field X-gal. It's related to lactose. The important thing is that when X-gal gets cleaved by beta-galactosidase, it releases galactose and this molecule which precipitates and turns blue. So we have a visual indicator of beta-galactosidase activity by placing in the medium, either in liquid medium or on a Petri dish, this substance X-gal. We know whether or not the cells have beta-galactosidase activity by virtue of whether the cells or the colonies turn blue. OK. So the first thing that we're going to do in this technique is to take some wild type E. coli. If we take a liquid culture of wild type E. coli and we plate them onto a tissue culture, a Petri dish that contains X-gal, this substrate that in the presence of beta-galactosidase will turn blue, will get colonies. And what color will those colonies be? Blue. These are normal E. coli. So they're able to metabolize the X-gal and produce this blue pigment. OK? Now, I'm interested in the enzyme, the gene that encodes the enzyme that carries out this activity. And the way that I'm going to get to it is first to isolate mutant E. coli. It cannot do this reaction. I'm going to try to isolate a mutant strain that's deficient in beta-galactosidase activity. And the way that I do that is to mutagenize the wild type E. coli. I can do this by physical methods or more commonly by just adding a chemical mutagen to the broth that the E. coli is growing in. I mutagenize the cells and then I plate them onto a plate that has X-gal. Now, most of the cells that got mutagenized will have mutated some other gene, not the beta-galactosidase gene, some other gene, or maybe no gene, such that what color will those colonies be? They'll be blue because they still have a functional beta-galactosidase enzyme. They still have a wild type lacZ gene. So most of the colonies will be blue. But what if the cell incurred a mutation in the lacZ gene that knocked out lacZ function? What color will the colony be? Not blue. It will be white. OK? And we're going to assume, for the sake of argument, that if you get a white colony it means that the cells have a mutation in this gene lacZ. That's actually not a safe assumption. It could have a mutation in some other gene, but we're not going to worry about that today. Just say that if we get a white colony we're going to assume that the cells have a mutation in the lacZ gene. So now we want to clone the lacZ gene. Our goal is to clone the lacZ gene. What are we going to do? What are we going to do? Well, I'll give you the first clue. We're going to make a library. We're going to make a library of fragments of DNA some of which will carry the lacZ gene. From what are we going to make that library? What will be our source DNA to make that library? Will it be these cells isolated from this colony or will it be some other cell? Do these cells have a functional lacZ gene? No. Do these cells? Yes. So you want to make a library from wild type E. coli. So that you have a bunch of plasmids that carry fragments of the E. coli genome some of which carry the lacZ gene. What am I going to do with that library? What am I going to do with that collection of clones? I'm going to transform it en masse all the different clones to a population of cells. What cells will I transform it into? Which bacteria? Would I put those ones into the lacZ mutants? And then I plate that transformation onto plates that contain amp. I need the drug to identify those cells that have picked up any plasmid because I don't care about cells that haven't picked up any plasmid so I use amp plates. And what else do the plates have in them? The indicator of lacZ activity, X-gal. So I'm going to take these transformants, which were derived from these cells, and I'm going to plate them out onto a plate. What color will most of the colonies be? Will most of them, raise your hand. Will most of them be white? Will most of them be blue? In fact, most of them will be white because most of the cells did not pick up a plasmid that carries the functional lacZ gene. Most of the cells picked up a plasmid that carried some other piece of the E. coli genome. OK? So most of the cells will not be, will not have functional lacZ activity, they'll be white. However, at some frequency a clone, a cell will pick up a recombinant plasmid that does carry the lacZ gene and that colony will turn blue. And that's the colony that we're now interested in because it has reconstituted lacZ activity. The mutant, the recombinant DNA has complemented the mutation. So I could isolate this plasmid and sequence it. And based on the sequence I might have confidence that indeed it is the lacZ gene. Remember I told you that there was an assumption built in here that this mutation really did affect the lacZ gene? And that's why the cells were white. That was an assumption. And to confirm that assumption we could look at the sequence of the clone that we isolated and figure it out, figure out whether it's the right enzyme. OK? So I find that teaching this is always a little confusing. I tried to simplify it today and go through it slowly. Hopefully you took notes and you can think about it on your own. It is relatively straightforward and it's a useful concept to understand that A) you could isolate mutations, and B) you can find the genes that were mutated by complementing those mutations through cloning. OK? OK. So now that I've spent a lecture and a half talking about cloning, I'm now going to tell you that cloning, while it was incredibly important and still is, has almost been superceded by another technique. It's not that we don't use cloning. We actually use it all the time. But it's been joined by another technique known as PCR which stands for the polymerase chain reaction. And any of you who have worked in molecular biology labs have been exposed to this because it is a totally pervasive technology. Everybody uses it. And it's not just in molecular biology labs. Forensic labs. Archeology labs. Anybody who's interested in generating large amounts of DNA from a small amount of DNA uses this technique called PCR. It was invented only a few years ago, but it's incredibly important. In fact, the guy who invented it, who was not a very well known scientist, won the Nobel Prize just a couple of years after he invented it because it became so powerful so quickly. It's a very, very simple technique. It's one of these ah-ha techniques that after it's been invented and described everybody says, oh, I could have thought of that. But, actually, nobody did until this guy, Kary Mullis. So I'm going to show it to you briefly on the board. And then we're going to go through a movie that shows it again. So hopefully you'll get how it works. Importantly, it relies on having some information about the DNA that you want to amplify up in large quantities. Again, the goal here is to amplify up a piece of DNA of interest to large quantities. This technique is so powerful that you can use a single DNA molecule to start. You can use the amount of DNA that you get from hair from a crime scene, for example. And that's mostly the way, you know. What is it? CSI Miami and stuff. They use this technique all the time. So you can use minuscule amounts of DNA. Even a single molecule is enough. But you do need to know a little bit of known sequence. So if you know a little bit of known sequence you can use PCR. So the first thing you do is to denature the DNA. And in this case we do that by heat. If you heat up a DNA molecule it will also separate from itself. And we typically heat it up to about 94 degrees. And the consequence of this is this double-stranded piece of DNA molecule will separate into two single-stranded pieces of DNA. And at that point we anneal, hybridize onto the DNA -- I'm not about to do another demonstration here. We anneal onto the DNA oligonucleotides, synthetic little fragments of DNA that are complementary to this known sequence at this end and at this end. So I synthesize a short oligonucleotide which will anneal to this strand at this position. OK? I should have written up the polarities because people often get confused. Here's the 5 prime end of this DNA molecule, the 3 prime end of that DNA molecule, the 3 prime end of that one, 5 prime end of that one. So if I'm thinking about the top strand here, the 5 prime end here, the 3 prime end here, and that little oligonucleotide, which in this context we call a primer, would have its 5 prime end here and its 3 prime end here. Thus, the arrow because this is the direction of DNA synthesis. If we're going to synthesize DNA off of this piece of DNA it's going to go in that direction. And then we likewise order up and anneal onto an oligonucleotide that binds to this strand of this sequence. And, again, to show the polarities, here's the 5 prime end. Ah, thank you very much. Here's the 5 prime end, here's the 3 prime end, so that the oligonucleotide primer has its 5 prime end here, its 3 prime end here. OK. And now these primers are sitting in such a way, annealed to a template piece of DNA with a 3 prime end facing in this direction, that are ready to be extended by DNA polymerase. Sorry. I'm getting confused about my colors here. You can't see them on the board? You can't see the blue. OK. All right. So the blue, sorry about this. The blue will now be orange. OK? And this one will be pink. OK? So I'm going to extend this pink oligonucleotide with a polymerization reaction to fill in this strand. And I'm going to extend the orange guy likewise to fill in this strand. OK? So this is accomplished by addition of DNA polymerase and the nucleotide precursors that are needed for DNA synthesis, which we abbreviate dNTPs. And if I incubate that in that way I'll get extension from this primer in this direction, extension from this primer in this direction. And what I've done here is to go from one DNA molecule to two DNA molecules. I've duplicated this piece of DNA in a test-tube. The beauty of PCR is that you then go through this process again and again and again and again. And each time you get an exponential increase in the amount of DNA. You go from one to two to four to eight and so on and so on and so on and so on. And in a relatively short time you can now have millions, billions or more copies of your DNA. OK? So that's the basic principle. And, again, we'll go through it in a little more detail in the movie. As I said, the technique was invented by Kary Mullis, an investigator at a company called Cetus. Kary Mullis is an interesting character. I don't have time to tell you the full stories of Kary Mullis. As I told you, he won the Nobel Prize. He's a real California kind of a surfer dude. Very laid back. So, anyway, you can use this technique for lots of applications, as shown here. It's been commercialized in many ways. We now have machines that will go through these various stages of denaturation, annealing and synthesis using a temperature regulated block that will go from 94 degrees to 68 degrees to 72 degrees to allow denaturation, annealing and polymerization around this circle many, many, many times. And if you do this, for example, 30 times, if you go through this cycle 30 times you make two to the thirtieth copies of your DNA, which is ten to the ninth DNA molecules, which is a ton. And that can be done in just a couple of hours using a machine such as this. OK. So let me take you through this movie. I'm going to cut it short because the process is gone through in great detail. The narrator is actually Paul Matsudaira who is a professor here at MIT. And Paul really sort of drags it on, but I'm going to -- Basically all he says is what I said, which is you take an individual DNA molecule for which you know sequences, and you run through this reaction multiple times. And each time you do you get a duplication of the DNA sequence. And your book goes through it, too. So I will get this thing posted on the Web. It's not on Monday's quiz so you don't have to worry about that. We can learn about it in the future. Maybe I'll fix it so that on Monday's lecture or Wednesday's lecture, Professor Sive who is giving that lecture can go through it with you. So sorry about this. I also wanted to mention briefly that the enzyme that we use to carry out the polymerization, the particular DNA polymerase that we use is isolated from what we call a thermophilic bacterium isolated from the hot springs. And that's important because we carry out this reaction at a very high temperature. You'll notice that the polymerization was done at 72 degrees. Now, your DNA polymerases won't work at 72 degrees but this organism grows at very, very high temperatures so its DNA polymerase can function there. And this, again, has turned into a cottage industry. There are hundreds of millions of dollars worth of this particular polymerase called Taq polymerase sold for this application. OK. So let's carry onto the next topic then. So what we've been talking about now is transferring DNA in the case of cloning or amplifying DNA for various applications. For some applications that's not good enough. For certain therapeutic applications, for example, taking human genes and cloning them to make therapeutic proteins or to do gene therapy you cannot do standard cloning. And the reason is that human genes are usually way too big. They have exons and introns, which you learned about, and they can be hundreds of thousands and sometimes millions of nucleotides in length. And that's way too big to fit into one of these plasmid cloning vectors and to efficiently introduce it to bacteria. And so for the purposes of generating clones from human genes we often use a technique called cDNA cloning. And I'll take you through this briefly. Recall that human DNA, human genes are broken up into coding elements that we call exons. And we usually number these exons from left to right, one, two, three. During the process of transcription a copy of this gene is made in the form of a precursor mRNA which has the same sequence. It has both the exons and the introns present. And then before this is translated into protein the information in the introns has to be removed in a process called splicing. And that produces a mRNA which carries the exons lined up next to each other. So the introns are removed, the exons are joined, and it's this mRNA that then gets translated into protein. As I said, this can be very, very large. But this is rather short. And so if we want to make a copy of a gene we can also make a copy of its mRNA. And that might be more efficient and more useful. And this is a technique called cDNA cloning. It was made possible by an invention at MIT, a discovery at MIT of an enzyme that was not known to exist, an enzyme which violated the so-called central dogma. The central dogma being that DNA is transcribed into RNA and that's translated into protein. David Baltimore discovered an enzyme called reverse transcriptase. Which can take the information in RNA and convert it to a DNA form. And he actually won the Nobel Prize for that. So this is the principle. You can take an mRNA which has polarity 5 prime to 3 prime. This mRNA that I produced up there, for example. You can anneal onto it an oligonucleotide primer. This oligonucleotide primer is made of DNA. It has a 5 prime end and a 3 prime end. And then you add this enzyme reverse transcriptase, which I'll abbreviate RT. Reverse transcriptase is unique in its ability to copy from an RNA template a DNA strand. And so you'll get a duplex which has RNA on the top and DNA on the bottom. You then get rid of the RNA. You hydrolyze the RNA. So all you're left with is that single strand of DNA. You now anneal on another primer, another oligonucleotide primer which now has a polarity 3 prime to 5 prime in this direction because this one went 5 prime to 3 prime in this direction. And then you add DNA polymerase. Which will extend from this primer and make a full duplex of DNA where both strands are made out of DNA. And this we call a cDNA clone, a copy of the mRNA. cDNA clone. And you can make libraries of cDNA clones from all the RNAs in your cells, for example. And then you can look, using various techniques, at different clones within that library for ones that function in different ways. So I want to take you through a couple of examples of how we use cDNAs in the context of gene therapy. This one comes out of your book. This is an application where there's an enzyme called TPA which will dissolve clots. It's called tissue plasminogen activator. And it's used now to treat people who've had heart attacks or strokes. So what was done was to make a cDNA copy of the TPA gene, introduce that into a plasmid vector with appropriate sequences in red and yellow to allow the expression of that gene in bacteria, transfer it into E. coli by transformation. Now, the E. coli will pump out this enzyme TPA. You can then purify that enzyme and inject it into this happy looking stroke patient to help dissolve the clots that it formed in the formation of the stroke. OK? So that's an example of genetic engineering one of our genes into bacteria to turn them into little protein factories from which you can purify the enzyme and hopefully mitigate the consequences of the disease. A second example, as opposed to putting in a therapeutic protein, you might want to put in a therapeutic gene. So the example here I'll give you is cystic fibrosis. I've mentioned to you in earlier lectures that cystic fibrosis is a genetic disease in which the individuals have a defect in a transporter, an ion transporter, so that in contrast to normal cells, here is a normal cell which has this CFTR transporter which will allow chloride ions to move in and out of the cell, as is a wild type individual. In the case of CF, that transporter is missing. That leads to an inability of the cells to properly regulate their water content, and this leads to disease. So what could you do about this disease? Well, why don't you just put the defective gene back in? We could make a cDNA copy of the cystic fibrosis gene, build it into a vector and introduce it not into bacteria but back into these cells. So we could clone a CFTR cDNA back into these cells. They would, in fact, pick up that DNA and begin to express it. They would make this protein. And that would allow them to transport chloride properly. And they might be ìnormalî. This actually works extremely well in the tissue culture dish. It doesn't work nearly so well in the context of a human being. Even though we can make viral versions of this, viruses that carry cDNAs, recombinant viruses, and I'll draw that up here. Using the same methods of recombinant DNA technology, I can make a recombinant virus, like a cold virus that now carries a piece of this cDNA for CFTR, and I could use that to infect a person who has CF. That's a person and they have CF so they're not happy. I could introduce into their nasal passage, they could breath in this virus that carries the gene. And if the gene, if the virus infected the cells of the lung, remember the problem occurs in the lung, maybe, if this were very efficient, the person would be happy, we could cure them. The problem is that this introduction of the virus is very inefficient in vivo, in the person. It doesn't work very well. You cannot get enough virus in to infect enough cells to correct the disease in the person. So although gene therapy is very attractive for many diseases such as CF, it actually hasn't worked very well. But there is one example of a cure using gene therapy. And I want to just end by telling you that story. To cure the disease you probably heard about, the disease that causes ìthe boy in the bubbleî syndrome, which is severe combined immunodeficiency, or at least one form of it, these individuals have a defect in an enzyme called ADA, adenosine deaminase. And this results in a very severe immunodeficiency. They don't have their immune cells. They cannot fight infection. So the kids have to stay inside protective chambers to avoid exposure to pathogens, the bubble. For a long time they really weren't well treated. They could be treated with having a little bit of the enzyme. I guess that's not shown here but on the next slide. If you add the enzyme back, even to their blood, you can get some protection. But, nevertheless, the kids did not do terribly well and often died from infections. Here's the disease mechanism. It's caused by a defective enzyme that normally breaks down adenosine. This leads to a buildup of a precursor, or rather a metabolite that kill cells of the immune system, and the individuals have immunocompromised state. If you can just hang on for one or two more minutes. So what's done here, in contrast to the failed example for CF where I said that it's very difficult to get the virus into the cells in the person, what's done in the case of this disease is to take the cells out of the person. And this is called ex vivo gene therapy where you isolate cells from the bone marrow of one of these kids, the stem cells that give rise to the cells of the blood system, and then you infect those cells in vitro with the virus that carries the cDNA of the ADA gene. You isolate those cells that have that gene and then you introduce the cells back into the person. And you can control that process much more carefully, much more efficiently, and you have a much greater concentration of cells that are doing the right thing now. This goes through it in some more detail. It's a version of a slide from your book so you can look at it. It's gene therapy. And I want to point out that although it can work, and it does work, it has one risk, namely that the virus that carries the therapeutic gene actually integrates into the DNA of the cells. And if it integrates into a gene that's important that integration could actually cause a mutation. So there's a risk associated with gene therapy. And, in fact, although this was successful, as you can see here, two kids with SCID, or actually a number of kids with SCID were cured through this ex vivo gene therapy approach, and now they can run around like everyday kids, unfortunately, in this first study of 11 patients, all of whom were ìcuredî, later two of the kids ended up getting leukemia. And they did because the virus had inserted itself, the genome of the virus had inserted itself next to a gene that when it becomes too active it causes the cells of the immune system to proliferate abnormally leading to leukemia. So although there was a benefit there was also a risk. In this case, families of these kids actually are willing to take that risk because it's such an awful disease. But you have to be aware that in all of these kinds of therapies there's a risk-benefit analysis that has to go on.