Flash and JavaScript are required for this feature.
Download the track from iTunes U or the Internet Archive.
Lecturer: Prof. Hazel Sive
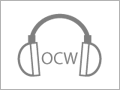
21: Cell Type and Position
What we talked about last time was formation of different types of cells in the embryo, different positions, different shapes. Am I on? And we talked about one of the overriding principles of all of this being the control of gene expression. I'll explore this a little more with a couple of slides in a moment. Let me just write some points. So we talked about the control of gene expression as being an overriding principle of how cells types are formed. We talked about the fact that cell types were formed in a stepwise fashion. And different parts of the embryo indeed in a stepwise fashion. Not all at once. And we talked about a combinatorial code -- -- of regulators that, together, specify different types of cells. So here we are to remind you talking about cell type in the formation module stepping through our board of life. And here are a couple of slides to remind you what we talked about in last lecture to bring you up to speed. You should look at the PowerPoint on the Web if you have not already. Cell type is defined by the proteins a particular cell makes. For example, red blood cells carry oxygen around the body because they make globin which is able to carry oxygen. The set of proteins that are made are defined by which genes in a cell are active, or expressed is the term you should know. And, therefore, which genes are active, also called the control of gene expression, controls which cell type forms. This triad is essential for you to understand what we're going to be talking about now and really for the rest of the course. So you should really get it. If you don't see me, go back and look at the previous lecture. And this lecture is going to also reinforce this triad. I pointed out last time that gene expression can be controlled at many levels. In fact, at any point from transcription, even from replication actually of the DNA through transcription, splicing, translation, export of the RNA to the cytoplasm, I haven't written up here, messenger RNA stability, protein modification, protein export. Any of these steps is along the way to getting the final gene product. And any of these steps can be controlled to allow the readout of the final gene product or not. I told you that along the way to becoming the final type of cell that a cell is going to become, it goes through a couple of steps. Or it can go through many steps, and we'll talk about that extensively today. Cells begin as uncommitted or naive. They don't know what they're going to become. They then become committed or determined to a particular fate, a position or a structure, but they may not look very different from that first set of uncommitted cells. And then they go on eventually to form so-called differentiated cells or the final cell type which has the function of that particular cell type. And so the point is if there is a stepwise formation of the final cell type. And that final cell type is directed through the action of regulatory factors that we termed inducers or determinants. There is nothing magic about these. Inducers referred to factors that were involved in cell-cell signaling. Determinants referred to regulatory factors that acted within a cell and were inherited by it. You shouldn't be trying to write all this down now actually because this is really review. And if you weren't at the last lecture, try to get some of it to wash over you and then go back and make sure you really know this stuff. And then as the differentiated cells form there is activation of a set of genes called differentiation genes which are the things that carry out the final cell function, the globin protein in red blood cells, the neurofilaments and neurotransmitters in neurons and so on. And we'll talk about this again as we go through today. OK. So this is not unrelated to what we are going to talk about today. Because what I want to do today is to explore with you how you get a particular kind of cell. And I want to use that exploration to underline some of the principles that I haven't yet covered as to how cell type is determined in the embryo. And the cell type I'm going to talk to you about is skeletal muscle. No, this is not the [UNINTELLIGIBLE]. OK. So the point today is to look at a particular cell type and how it forms. The type we've chosen is skeletal muscle. Skeletal muscle is one of three kinds of muscle in your body. The other two being cardiac in your heart and smooth muscle around your internal organs. Skeletal muscle acts in a voluntary fashion to allow movement. It's an extremely complex structure. The final form of skeletal muscle includes 200 proteins which have the extraordinary property of contractility in response to nerve input. I'm not going to talk about the contractile process, although it's fascinating. And you're not going to be tested on this for the physiology of contractility either. But if you're interested in this, I would suggest you look at Chapter 47 in your book which talks about this quite nicely. Skeletal muscles are arranged in large groups of fibers. And each muscle fiber is a multinucleate cell, is a giant cell that consists of many myofibrils or myofibrils that are together in a sheath and are surrounded by many nuclei. So skeletal muscle forms from the fusion of many mononucleate cells to give a multinucleate syncytium. And during the process of skeletal muscle formation there are a number of steps that one can delineate. One can talk about a kind of cell called a myoblast which is a single mononucleate cell -- -- that then fuses to form, through many steps, something called a myotube which is multinucleate. And these various myotubes come together to eventually form a muscle fiber. Muscle is obviously an extremely important cell type for movement. All of the meat that you eat, if you're a meat eater, is muscle, essentially a skeletal muscle. And there are many diseases that are associated with abnormal skeletal muscle. Muscular dystrophy, for example, is a deficit of one particular protein in the muscle, affects one in about 3,000 boys. OK. So the myofibril is a very beautiful construction of many proteins organized in a very ordered structure. And the deal is that these proteins slide relative to one another during contractility. And all of these bands of proteins slide in an ordered fashion relative to one another as the muscle contracts. It's an extraordinary story in physiology itself. And I regret I don't have time to go through it with you. But I want to use this rather as an example of a cell type and to explore with you how the cell type forms during development. So the story I'm going to tell you has to do with a particular transcription factor called MyoD. MyoD, shown in green here, no, not shown in green here. That's the DNA double helix. Shown in red here binds to a particular sequence on DNA, its target site. It binds as a dimer. And it acts to activate the transcription of genes required for muscle formation. OK. So MyoD is a transcription factor. And, as I'll tell you a moment, it gives rise to the determination of skeletal muscle. Now, I'm going to tell you a few things about this that are general principles of how this transcription factor works and then I'll go through some slides with you. MyoD is both necessary, although I'm going to clarify that in a moment, and sufficient for muscle determination. This is a dyad of words that is very important, necessary: required for, sufficient: enough to. And whenever you're thinking about gene function, this is the dyad you want to think about. It may not be a gene, and then I'll tell you in a moment. I'm going to put a caveat here with respect to the necessary, OK? But necessary and sufficient is one of these litanies that you want to ask yourself when you're thinking about regulatory factors. The other thing that I want to tell you is that MyoD is a member of a gene family. What's a gene family? A gene family is a group of genes with similar structure and often similar function. This gene family that MyoD belongs to is called the MRF gene family, which stands for muscle regulatory family. And there are four members. And these members share some function with one another. And that leads us to another term which is the term redundant. MyoD is an extremely important gene. And if you make knockouts in mice there is some phenotype, but they have muscle. And it's not until you make a gene mutation in another member of the MRF family that you see mice that don't have any skeletal muscle. So redundant in this case refers to, or in all cases refers to shared function. It can also refer to, and does in this case, it can also refer to alternate pathways. So the notion that we have is that we're starting here as an uncommitted cell and we're going to end up over there as a differentiated muscle cell. And we can get there by walking straight this way, but we could also get there by walking up and out the back and all the way around and to that same spot in front. And both of those would be bona fide pathways that would get you from point A to point B. So sometimes there can be alternate pathways that will get you along the path of forming particular cell types or even carrying out some kind of biochemical function. OK? This is a general principle of biology. So let's look at this in a bit more detail with some slides. The notion is that MyoD binds to the promoters of many different genes expressed in skeletal muscle, together with other transcription factors, some of which may be common to all of these genes and some of which may be different. And it activates their transcription. And you can see I haven't drawn the genes in a really accurate way. These are just representations. There's no double-stranded DNA meant to be implied or not implied there. MyoD is sufficient to activate the skeletal muscle program. And this is a very exciting finding about 15 years ago when it was shown that one could take the MyoD gene, and you actually put it in a virus, and then you can infect a group of cells that normally wouldn't become muscle with this virus. And lo and behold the virus that is expressing the MyoD protein will turn these cells into skeletal muscle. And this was the first example of a regulatory protein that could take one cell type and turn it into another cell type. And MyoD and the other MRFs are very good at doing this. They can, for example, take brain cells and turn those into muscle. They can really take most cell types and turn them into muscle cells. So MyoD is sufficient to convert non-muscle to muscle cells. And this is actually particularly extraordinary because, as I showed you in a one line schematic on this board, forming skeletal muscle is a multi-step program. You start off with these cells called, they call them myotome cells, and then you get these things called myoblasts which are dividing. The myoblasts start to align in arrays. And then the cells fuse with one another, which is really unusual for cells. I mean it's a really kind of weird cell type. And you get these long tubes that have got thousands of nuclei in them. And then the tubes start to make proteins that align themselves in these very ordered ways that allow them to slide relative to one another, and you get this multinucleate myotube that has contractile function. And what's really cool about MyoD is that it can start this whole process which then follows one step after another. So it's been termed a master regulatory switch because it can activate a whole cell type specific program. Now, in contrast, and this is what I was alluding to on the board, knockout or removal, a genetic mutation of MyoD does not remove skeletal muscle from mice. So this is a micrograph of muscle. You can see the ordered myotubes from a wild type mouse. This is a MyoD null mouse. And there's perfectly fine skeletal muscle there. It's a little, there's a little less of it, but it's still there and the mouse is viable. However, if you go in and you make a double mutation in the MyoD gene and another member of the family called Mif5, there is no muscle at all in any region, or no skeletal muscle at all in any region of the body. The other muscle, the heart muscle and the muscle around the intestine, the smooth muscle is fine, but the skeletal muscle is completely absent. So we can say that the MRFs are necessary for muscle formation but that MyoD is a member of a redundant gene family. And that seems to be the rule in biology, that genes come in families, and very often one can take over the function of another when one of them is mutated. And that serves as a kind of fail-safe for making sure that development works properly. OK. So here comes something to think about that I alluded to before spring break. I remember this. Don't know if you do. MyoD is, in the entire embryo the MyoD gene is only expressed in the future skeletal muscle. This is a picture of a frog embryo. And you can see these stripes along the back of the frog. These are regions of the embryo called the myotones. They're part of the somites that I'll talk about. And this is what's going to become the future skeletal muscle. And this is where MyoD is expressed. And, brilliant, only there. And now this is a chicken embryo and you see the same thing. MyoD is expressed in these stripes along the back in the future myotones of the somites. It's also expressed in the future limbs as the muscle of the limbs is starting to form. And so you're going to ask me, or you should, how does MyoD get activated just in the future skeletal muscle? Because in a way this is a copout. I've told you MyoD activates the skeletal muscle program, and it's only expressed in cells that are future skeletal muscle. Well, how does it know to be expressed just in this one region of the embryo? And to answer that question we have to actually go back to the dawn of time to fertilization. And I'm going to take you through some of the steps that will get MyoD expression into this particular pattern that will allow the skeletal muscle to grow out in just the right places of the embryo to give just the right amount and the right position of skeletal muscle. OK. So. And to do that we are going to pose some questions. And I'm going to start by telling you something and then pose a question. If you look at an embryo, you could look back at that chick one if you wanted to do or you can look at this fish. Here is your fish fillet, your skeletal muscle, and it is on the so-called dorsal or backside of the embryo. So last time we talked about dorsal and ventral as two of the so-called axis or positional coordinates in the embryo. And the muscle arises from a region that is termed dorsal which is the back. OK? So you might ask me, how do you know this? And I'll tell you how I know this. You should ask me how do I know this. OK, so I'm going to. Having put questions into your mouths, I'm going to tell you how you know during the development of an organism where a particular cell type is going to arise from. And the technique I'm going to tell you about is something called fate mapping. So let's begin with the sentence muscle, and I'm talking about skeletal muscle when I write muscle, arises from the dorsal or the back of the embryo. OK? And so how do you know this? How is this known? And it's known through use of a technique called fate mapping. What is fate mapping? So this is very interesting. The idea is to take a very early embryo, I've shown you here a fish embryo, and to inject one or a few of its cells that you can distinguish by some kind of morphological, obvious indicator from the rest of the embryo. And to inject those cells with a dye. So here we've used a fluorescent dye. And we've put that dye near the site of the embryo that's got this bump. OK? And as the embryo develops the cells will inherit that dye because it's a non-diffusible dye. And that dye will be inherited. And in the later fish, we're looking down now onto its brain, you can see that the whole front of the brain is outlined with these green fluorescent cells. And what this tells you is that the cell you injected initially has gone on to give rise to this whole front region of the brain. OK? So fate mapping tells you what cells will become. All right. I know it's on the slide, but it's important that you understand this because it is distinguished from something I'm going to tell you later. So you can do this for skeletal muscle. And I'm going to talk about frog development because so much is known. So here is a frog at the 32 cell stage. And one can see, because of the size of the cells and because of pigmentation differences I'll show you in a moment, which side of the embryo is what. And I've injected a cell here that's called the C3 cell, all these cells have got different names, with dye. And later on you can see, in this thought experiment, that the somites are labeled with the blue dye. So the C3 cell, and actually the one on the other side of embryo, are going to give rise to skeletal muscle. OK. So the reason that I wrote on the board what was on the slide already is that it's really important to distinguish what cells are going to become from when they've made the decision to become it. So the next thing we want to know is when cells decide what they're going to become. And I can rephrase that by saying when is the fate decision made? And fate mapping doesn't tell you that. All it tells you is what a cell is going to become, not when the cell has decided to become that thing. For this you have to do some other assay, and you do assays that are generally called determination assays. And I'll show you an example of one such assay. So here's our 32 cell stage frog embryo again with the C3 cell labeled. And one can go into the embryo and dissect out that cell. Frog embryos are about a millimeter in diameter. And you can use very fine glass needles to tease out that cell from the embryo. And you can grow it in a very simple culture method with just some saline solution and the cell will grow fine and divide. And you can ask whether that cell goes on to make skeletal muscle. OK? Does it know that it's going to be skeletal muscle? And the answer at this stage of development is no, it doesn't. So if you remove the cell, grow it in culture, it goes on to become these things called fibroblasts which are cells that spread out in the dish. They don't fuse. They don't make all these muscle proteins. And so the cell, although it's destined to become muscle, has not yet made the decision to take that leap of fate. However, if you wait a few hours, and we're actually on your handouts now. If you wait a few hours and you go and you remove the cells that have arisen from C3, which are divided now. They're still labeled and you can distinguish them because it's a very powerful dye and doesn't diffuse. So you can remove that same group of cells a bit later. And you can then grow those cells in a culture, in a simple saline solution. And lo and behold they go on, after some hours, and form multinucleate myotubes. They go on to form muscle. So somewhere between the 32 cell stage and the stage of a much older embryo of many hundreds of cells, the cells have made the decision to go onto their particular fate. So something has changed in terms of the regulatory genes that they're expressing, presumably, that allow them to go and become muscle. OK. So let's break this down a bit more. And let's ask the question, as to what inputs are required to turning on MyoD activation in a more defined sense? And the first thing I'm going to talk about is how the embryo knows where dorsal is. OK? So dorsal determination. And what I'm going to tell you is a very interesting tale of determinants, of cell autonomous regulatory factors that are specifically required to tell the embryo where the future dorsal or backside of the embryo is going to be. And I'll tell you that these determinants act to stabilize a particular protein that is a transcription factor, and this protein is called beta-catenin. And beta-catenin, once it's stabilized, so it is a transcription factor, goes on to activate a set of genes that are dorsal specific genes, but not MyoD. We're not there yet. Gene. G. I cannot spell, can I? OK. So let's discuss this using your handouts. This is a movie that I was showing you at the beginning. Woops. Let's show it to you again because I want to point something out. All right. This is a four cell embryo. Maybe it's going to be an eight cell embryo. Do you see how these embryos have got some, two cells that are kind of darkly pigmented and two cells that are lightly pigmented? Yeah? The lightly pigmented cells are the ones that are going to be the future dorsal site of the embryo. OK? That's where the muscle is going to come from. And these cells on the other side, on the ventral side of the embryo, that's where the belly is going to come from. Now, how does this happen? Well, it happens, and I've augmented the diagram on one of your handouts a bit so you'll have to add a circle. It happens because of movement of these purple dorsal determinants. And these purple dorsal determinants, as I'll show you, move within the newly fertilized embryo. In fact, within about 16 minutes after fertilization these dorsal determinants have moved in the embryo and have told the embryo where the future dorsal side is going to be. And the way they move is that, in fact, the early egg and the zygote consist of two kinds of cytoplasm, an outer sphere of cytoplasm that's kind of gelatinous and an inner sphere of cytoplasm that is more liquid. And in this outer gelatinous cytoplasm sit these purple determinants. And when fertilization takes place these move by a good angle, at least 30 degrees relative to where they were, and relative to this inner liquid cytoplasm, which is why I've got this doted equator there to indicate that there is a relative movement of these determinants. OK? It's not the whole embryo that's moving. It's just part of the embryo that's moving relative to the other part. And this movement of determinants defines the dorsal and the ventral side of the embryo. OK. Here's a movie to show you that. OK. So this is a movie that is about the first 20 minutes of fertilization. And you can see here pigment moving from one region to another region. Now, the pigment is not the determinants. It just moves with the determinants. OK? So this is an indication that there is stuff moving, actually, from the vegetal pole region up towards this so-called animal pole region. So the terms animal and vegetal pole indicate two different sides of the radially symmetric egg. The animal pole is pigmented. The vegetal pole is not. And they're just useful landmarks. And, again, this is a frog embryo. Things may be similar in humans, but we understand much more about frogs. So that's why I'm going to tell you how things work in frogs. OK. So there is a manifestation of these dorsal determinants moving. These dorsal determinants move by a really fantastic process. They move on microtubules. Remember microtubules? What part of the embryo, what part of the cell were microtubules part of? Anyone remember? Dredge back in your memories. I heard something. Yes? Thank you. Cytoskeleton. Yes. See me for a frog afterward. So the microtubules are part of the so-called cytoskeleton. They are large rods of proteins, polymers of proteins. And when the sperm enters the egg it brings with it a centriole that is a microtubular organization center. And what it does, in this series of three pictures, is to take this mass of red microtubules that are oriented in every which way, and it organizes them so they form these beautiful parallel tracks of microtubules in the outer cytoplasm or between the outer and inner cytoplasm of the egg or of the zygote, of the fertilized egg. So the sperm nucleus or the sperm, excuse me, the sperm centriole is organizing this microtubular array. And it's on these tracks of microtubules that these determinants move, kind of like train tracks. And I'll tell you in a moment, it really is that way. So, oh, it didn't quite work. But OK. So here is a zygote and here is this protein I've told you about, beta-catenin. Now, in the zygote beta-catenin is phosphorylated. And that renders it unstable for various reasons. And it's also cytoplasmic. OK? With the action of the determinants I've been telling you about, by about the two to four cell stage, so very soon after fertilization the beta-catenin on one side of the embryo, the side where these determinants are has been dephosphorylated. So there is a post-translational modification. Dephosphorylated. And that renders it stable for various reasons. It's no longer subject to protein degradation. It also allows it to enter the nucleus. It allows it to act as a transcription factor. So these determinants are changing the stability and the protein structure of this beta-catenin transcription factor. Beta-catenin is really an important protein. This is a wild type frog embryo. Two wild type frog embryos. The head, tail, here's the eye forming. In embryos that have been depleted of beta-catenin you get these kinds of blobs that have no dorsal structures at all. They've got no muscle. They've got no nervous system. Nothing that would normally come from the dorsal side of the embryo. So what is the identity of these dorsal determinants? And, again, this you have partly on your handouts. And the identity of the dorsal determinants ergo is something that stabilizes beta-catenin and dephosphorylates it. And what these are believed to be presently are two proteins called GBP and dsh. OK? You don't need to know the names. It's the principle that's important here. But what's very interesting is that GBP and dsh proteins bind to another protein called kinesin, and kinesin attaches or is part of the microtubule system. So you get these determinants attaching to the microtubules, and as these microtubules polymerize and have a rotation associated with them, it's not quite clear they rotate at this point, this GBP and dsh are associated with the microtubules. And then these two proteins, GBP and dsh, also called disheveled, inhibit another protein called GSK3 which is a kinase that phosphorylates beta-catenin and renders it unstable. So you're getting here dorsal determination. You can go think about this more clearly. Dorsal determination because you are inhibiting an inhibitor. All right? Complicated? Yes. Beautifully understood? More or less. This is really the most beautiful example that we know of how you get determination of a particular region of the embryo. OK. So one step. We probably have about 20 steps to go. And clearly we're not going to cover them all so I'm giving you a little meander through the number of steps that are involved. So another step informing muscle, that I want to tell you about, is forming a particular kind of cell from which the muscle will arise. So not only does muscle arise from cells that are dorsally located. Muscle also arises from a cell type called the mesoderm. The mesoderm is part of a system of cells that are the earliest known cell types in the embryo. And these are the germ layers. And the germ layers consist of mesoderm, and also of two other cells types called ectoderm and endoderm. OK. And these cell types were discovered a lot time ago. They're not differentiated cell types but they do have different properties. They are stepwise along the way to becoming different things. The mesoderm, shown in red here, gives rise to the muscle, blood, kidney, heart and various other things. OK? The ectoderm, for example, gives rise to all of the nervous system, and the endoderm to the gut and the lung. And they get their names from their position in the early embryo. The endoderm is on the inside, the ectoderm on the outside, and the mesoderm between the two. OK. How does the mesoderm form? The mesoderm forms through another complicated system. It forms through the action of a transcription factor called VegT. And this is a maternally expressed transcription factor. So we can distinguish maternal and zygotic effects, genetically and in development. VegT is maternally expressed. That means it is present in the egg. VegT in term activates in just one region of the embryo another gene called nodal. It's actually a set of genes. Nodal is a secreted factor. It is actually a ligand and, therefore, an inducer. And it is zygotically expressed. So another principle, "zygotically X" for expressed, and "maternally X" for expressed. OK. This is one your handouts. So we're talking now about the same time of development that we've been talking about but a different system that is working in parallel. Here is an embryo. And I've shown you between the 200 and the 500 cell stage that has got this VegT transcription factor present in the nucleus. It's a transcription factor. And you can find a concentration gradient of this thing. In one part of the embryo at this equatorial region there are low amounts of it. Towards the vegetal pole there are high amounts. And where you have this low amount of VegT you get a band of cells that's going to become mesoderm. This mesoderm then goes on to express this nodal gene which is a secreted ligand. And, for those of you who are familiar with this, it's a member of something called the TGF-beta family. So this gives you another set of cells to deal with. And what I'm going to tell you now is that to think about where the skeletal muscle comes from we have to superimpose the two things I've told you about becoming dorsal and becoming mesodermal. So the next thing we need to do is to talk about the dorsal mesoderm. And we can write an equation here where beta-catenin plus nodal makes dorsal mesoderm. And I'll tell you that this dorsal mesoderm has a very special property and a special name. It's called the organizer. Is the dorsal mesoderm the future skeletal muscle? I'm sorry to tell you it's not really. There's another step involved. And I will tell you in a moment that the organizer is going to induce the precursors of the skeletal muscle. So let me write this down just to give you a sense of where we are. OK. So let's move on here. And let's define dorsal mesoderm by an equation. And, in fact, you can define this whole process by an equation. But, of course, the genes that have to be expressed and so on have to be expressed in a specific temporal order. So it's not a simple linear equation. It's got a time component, too. OK. So here we have beta-catenin on the dorsal side. And we have mesoderm defined by nodal signaling in a band across the equator. And if we superimpose them we get a region where both of these factors are in the same group of cells. And where both of these factors are in the same group of cells we get a special type of mesoderm formed called the dorsal mesoderm or the organizer. The organizer is a very famous piece of mesoderm. And it is famous for this reason. This is a very old experiment that was done in the 1920s where one could take a piece of donor tissue from a donor embryo, that is the future organizer, and move it to a host embryo, and put it on the side of the embryo where the organizer wasn't. OK? So to put it on the ventral side of the host embryo. And when this was done, to the surprise of the investigators, because they really didn't do the experiment for this reason, they found that they got these conjoined twins. Conjoined twin embryos. Here's your host embryo. And this is a second embryo that's formed. And you could look at see where that piece of transplanted tissue went. And when you did you found it was in a strip along the back of the embryo, but most of the second embryo, the conjoined embryo came from tissue that was the host embryo tissue. In other words, this piece of transplanted tissue had organized or induced a second embryo, including all the skeletal muscle. OK? And including a head and a brain and so on. This is a very famous experiment, very famous piece of tissue. And for this experiment the Nobel Prize was awarded to Hans Spemann in 1935. Here are conjoined frog embryos made by transplanting organizers. You can see they have two heads and they're joined at their tail region. What about humans? Well, the same thing is true in human embryos. Usually identical twin embryos will split somewhere between the two cell stage and a very early stage where there is something called the inner cell mass. And you get two identical embryos made and you get completely separate embryos made. Sometimes, very rarely, you get incomplete splitting of the embryo. And the organizer is split in two in a human embryo, and you land up with human conjoined twins. This is very rare. The survival of conjoined twins is very rare. There are some instances, and I've put on your handout a diagram of, these are actual girls who had been diagramed that were just like the frogs, with shared heads, with a shared trunk and separate heads. So the processes in frogs, as far as we know, are very similar to those in humans. Now, what I'm going to do because I have very interesting stuff I'd like to finish this off, finish off here, but I see time is creeping on, is to end off with a teaser. And to tell you that the final step in getting MyoD on is to activate these things called somites. And I'm going to take five minutes at the beginning of next lecture to complete this and then we will move onto our next topic. So thank you.