Flash and JavaScript are required for this feature.
Download the track from iTunes U or the Internet Archive.
Lecturer: Prof. Hazel Sive
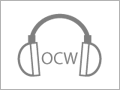
23: Stem Cells
Related Resources
Biology terms (PDF)
Differentiation. For someone who didn't see the board. Yeah? Yeah. So what a cell will become eventually. And differentiation is the process by which it becomes that. OK. Good. So these terms are essential for you to know for today's lecture. And if they are a little murky for you then you should really make sure they become clear. OK. I'm going to talk to you today about stem cells. This is the how-to module, our second how-to module. We have two lectures, stem cells and cloning. And what I want to do today is to go beyond the media, beyond the hype and tell you about stem cells, what we really know and why there is such a fuss about them. And to put it in perspective of where you are in the course, in our game board of life we're moving right along past foundations up through formation and now into our second how-to module. So let's start with a question. And you have a handout. If you do not have a handout, Shamsah, could you be mail person? And on the other side it looks like we need another mail person. You will realize that I typically do give you a handout of the most important slides so that you don't have to grapple with things and that you don't, in fact, have to print out the PowerPoint handout before you come. So you should always just check. OK. So what is a stem cell? Well, a stem cell is something that has two important capacities. Firstly, it can self-renew. That's a very popular term. It can make more of itself. And, secondly, it can give rise to a number of different, one or more different cell fates. So it can give rise -- -- to one or more differentiated cell types. Schematically, I've represented it for you in this way. So here is something that's called an uncommitted stem cell. And you should be familiar with the term uncommitted, naive and so on. And this uncommitted stem cell can undergo cell division to make more of itself. And it can also go on to become something that's termed in the field a "progenitor cell". It's just a term. It means a cell that is going to give rise to something else. And I've depicted it here as a determine progenitor. It knows what it's going to become. And this term uncommitted stem cell, the uncommitted part is a little misleading. I'll talk about that more in a moment. This determined progenitor then goes on to give rise to a whole bunch of one or more, actually, differentiated cell types. And the number of cell types that a stem cell can go on to give rise to is a measurement of its potency. That is the definition of the term potency. So this is the notion of the stem cell concept. You have this. It's the first figure on your handout. And you'll find this in many newspaper articles and books. So what's so special about this and why are there newspaper articles and newspaper articles and newspaper articles and television programs and covers of Time Magazine about stem cells? What's the hype? Well, the deal is that it is believed by some that stem cells are going to be some kind of universal repair kit. That somehow because stem cells can give rise often to differentiated cell types and make more of themselves that they are in a position to repair parts of the body when the body needs repair. You have a heart attack, you throw in some stem cells, repair the heart, you have a brain lesion, you throw in some stem cells and you repair the damage. And if you go through the newspapers, as I did very, very briefly, it's very easy to find many, many different headlines. One of the big ones last week, I'll talk about this at the end, is that the Massachusetts House passed a law expanding the use of stem cells in research. Hopkins is beginning human trials with donor adult stem cells to repair muscle damage from heart attack. This is an enormous amount of interest in these cells because of this notion of universal repair kit-ness. There are also advertisements. The Stem Cell Bank and the Cord Blood Registry would love you to pay them to save your child's umbilical cord stem cells, or the blood cells from a newborn's umbilical cord in the possibility that you'll use these later on to save the life of this child or somebody else. And the other reason that stem cells have got so much hype is that, as we'll discuss towards the end of the lecture, they involve the use, or are likely to involve the use of human embryos. And that's a real fire point. But let's step back and let's talk about stem cells and biologically what are these things and what do you need them for? And the thing we have to consider is the question of organ maintenance. Now, I've told you several times, oh, you see, it popped up. There you go. We have to give these frogs a moment to pop up. OK. That must have been somebody's frog that popped up. I've told you several times that our bodies consist of about ten to the twelfth cells. That's a lot of cells. But it's actually much worse than that because it is not the same ten to the twelfth cells that you started with, once you got there, that you keep throughout your life. The cells in your body are constantly dying and are constantly being replenished. And, in fact, if you look at your ten to the twelfth cells, over the course of your lifetime you will make many times ten to the twelfth cells. You're not going to replace every cell in your body because some of them life forever, but for many populations of cells in your body you will replace those organs completely. OK? So we're talking about making lots and lots of cells. And the issue really is one of organ and tissue maintenance. And if one considers how organs and tissues are maintained, they fall into three categories loosely. Those that have a high turnover rate where cells do not live for very long and need to be replenished rather frequently to maintain the size and function of the organ. Those organs that have a low turnover rate and those that are said to be static. Organs with a high turnover rate include, as I'll tell you a moment, the blood, some of your hair. Organs with a low turnover rate include things like your liver and pancreases. And organs that were thought to be static, but in fact that may not be true, was the nervous system. And now we know that that's not true. So let's talk about how we get to these terms. High turnover. Low turnover. Let me just write it out. Turnover. And static natures of organ maintenance. And the way we do this, or the way this has been done is by an assay that's called a pulse-chase assay. And a pulse-chase assay, and this is the second slide on your handout, goes like this. In a cell population one can add a nucleotide analog bromodeoxyuridine. It's uridine but it's incorporated into DNA because it's got that deoxy there. And bromodeoxyuridine is a nucleotide that is incorporated and can then be detected using various stains. So you can tell which cells have incorporated BrdU by staining them appropriately. So you can tell which cells are dividing, which cells have gone through S phase by whether they are labeled or not. And if you give a cell population a short pulse, if you give BrdU for just a short time, this is called a pulse, it gets incorporated into the DNA, and then the BrdU is gone. And so you label your cell population. And then you follow the cells over a long time without any added label. And so the only cells you look at are the ones that were labeled during this pulse period. And if you do that, you see that your initially labeled cell population lives for a while, and then the cells start to disappear because they die. And you can monitor the half-life a population by counting how long cells live for. OK? So this is a pulse-chase analysis. And this will give you the cell turnover time of a particular organ. And this is very useful in trying to figure out whether cells have a high turnover rate or a low turnover rate. Now, you can do something else with this. You can look at your labeled cell population, and you can actually look not just at the numbers of cells but you can look at what those cells become. And sometimes your initially labeled cell population will be the final form, the differentiated state of the cells, but sometimes it won't. And then it's very informative to follow that labeled cell population and see what they do. This is your third handout, your third slide on your handout. And if you follow them you might see that this labeled cell population, during the chase, differentiates into something. And that's very interesting. And then later on those cells will die and you'll be able to measure a half-life as previously, but this is very interesting because it tells you that this labeled cell population was not the same as the final fate of the cells. And it implies that there is some kind of precursor cell, some kind of progenitor in your labeled cell population that's giving rise to the differentiated cells. And it implies that these initially labeled cells either are stem cells or are progenitors derived from stem cells that are responsible for repopulating a tissue as the tissue or organ needs to be maintained. So these are two assays that are very useful and are used to, have been used to define high turnover organs. So what are some of these organs that have, or tissues that have high turnover? In fact, they've been used, these assays have been used to define many tissues, those with high and those with low turnover. But it's the high turnover ones I want to talk about. The red blood cells in your body have a turnover time, half-time of about 120 days. And if you do the calculation, because you have a lot of red blood cells, you're making more than a billion cells a day. OK? As you are sitting here, you are probably, during the course of this lecture, making several million new red blood cells. Your intestine, some of the cells in your intestine that are responsible for food absorption turnover every three to five days. Your skin cells, the epidermis of your skin turns over with a half-life of about 14 days. And your hair, depending on where it comes from, turns over with a half-life of about 14 days to about four years. And the sense in many of these organs, and I'll go through this a bit more in a moment, is that this high turnover and the replenishment of these cells as they disappear is driven by a stem cell population. And I'm going to abbreviate stem cells SC. And I'm going to put forth the hypothesis that stem cells replenish these organs. Now, in some cases you can actually look at the organ and you can do your pulse-chase, just like I told you, and you can see that this must be so. And an example that we've talked about previously, not in this context but previously is the testes, the spermatagonia are the cells sitting right at the edge of the seminiferous tubule, which we'd label with BrdU. And as you do your chase you would see those cells as labeled cells moving towards the lumen and differentiating into the stem cells. OK? And so you can actually see those cells giving rise to the differentiated cells. Another tissue where this is very beautiful is in the epidermis of the skin. So the epidermis of the skin comprises multiple layers stacked up on top of each other. And as you get towards the outside of the epidermis, the cells in the outer layers are dead and they form the layer that we feel as skin. But, in fact, there are a lot of cells that are living underneath the outer layer. In the very deepest layers of the epidermis reside a dividing population of cells that are, that include the stem cells, and I'll show you an assay for some of this in a little bit. These dividing cells, if you follow them, give rise to the cells in the layers above. So they either are the stem cells or they are progenitor cells that are replenishing the epidermis. Now, in the blood, I told you that you can do a pulse-chase assay. And you can very accurately measure the half-life of red blood cells. And it's clear that red blood cells must come from some precursor. In ourselves, in most vertebrates, in many vertebrates red blood cells do not have nuclei so they cannot divide themselves. And so there must be some kind of progenitor. But the progenitors of red blood cells and, in fact, many other cells in the blood lie deep within the bone marrow, the cavity of the bones. And so you really cannot observe them well to come to the conclusions that I've just given you about the testes and the epidermis of the skin. So you have to do some kind of other assay to figure out where these cells are coming from. And over the years a huge body of data has given rise to this notion. In the bone marrow of the long bones, and some of the shorter bones, too, there is some kind of pluripotential or pluripotent hematopoietic stem cell, that I've abbreviated HSC. And this HSC gives rise, through a series of progenitors which then go on to differentiate, into all of the cell types that are found in the blood. The red blood cells. The so-called white blood cells. The immune cells. A huge spectrum of differentiated cell types. And this hematopoietic stem cell has been very, very useful in helping people who have got blood associated illnesses in that it can, after removal of diseased blood cells, it can repopulate the tissue and help people get better. And it's done so, and I'll go through how this is done both in an assay sense and point out that this is exactly what's done in humans, it's done so in a bone marrow transplant. So in assays, let's just write down a couple of things here. I've told you one assay already for stem cells. OK? So assays for stem cells ask how do you know if you've got a stem cell? Well, you can do that. You can ask this using the pulse-chase by direct observation with your pulse-chase. You can also do it by a repopulation assay. And the notion in a repopulation assay is that you try to restore a particular cell type by putting in what you think is the precursor of that cell type. Now, in order to do these assays you usually have to get rid of the normal cells that were there so that you can assay your repopulation. OK? Otherwise, the normal cells can out-compete the cells you're putting in. OK? But that's an aside. So what is a bone marrow transplant? The notion here is that one takes an animal, and this is done in humans, and you irradiate the animal so that you destroy all its bone marrow. This will eventually kill the animal, OK, because you cannot live without a hematopoietic stem cell and without all the cells that derive from that. And so, although this irradiated animal would die, you can rescue it by injecting normal bone marrow. You inject it into a mouse in the tail vein, into people it goes into a central line that goes into your blood system. And then those bone marrow cells, it's extraordinary, know where to go. They home to the bone marrow and they repopulate it and they make again the hematopoietic stem cell and all the lineages that come from that. Or they are the hematopoietic stem cell, they maintain themselves and they give rise to all the other lineages. One of the criteria that I told you about stem cells is that they need to be self-renewing. You can ask whether or not these stem cells are self-renewing in an assay like this, where you take a mouse that you've rescued by bone marrow transplant, and you can ask whether it can serve as a source of marrow of hematopoietic stem cells that will rescue another irradiated mouse. In other words, you could have rescued that first mouse just by giving it a whole spectrum of almost differentiated red blood cells, white blood cells, immune cells and so on. OK? In order to test whether you actually rescued it by giving it a stem cell, you can ask whether or not you can take this rescued mouse and use it to rescue another mouse. And, in fact, you can. And this is consistent with, not unequivocal, but it's consistent with a sense that you have generated more stem cells in the rescued mouse that can go on to rescue another mouse. Another set of experiments that has been really pivotal in understanding stem cells is to try to purify the stem cells and to assay what are these pure stem cells. And part of this is asking how many cells do you need to rescue in a repopulation assay? OK. And so let me make a note here. That when it comes to stem cell isolation the overriding conclusion from many assays done by many labs is that stem cells are rare and they are hard to find. In this experiment, which is number five on your handout, you can assay for how many stem cells you need to rescue a mouse by removing bone marrow from one mouse and staining that bone marrow, if you can, for a stem cell marker. Now, what is a stem cell marker? OK? If I have a stem cell marker I would be able to go and pull out the stem cells. Well, there are no markers. A marker could be a protein or an RNA that is expressed in a particular group of cells that you suspect are the stem cells. OK? This is very much an experimental-driven field. There are markers that always appear, proteins that are always there, antigens on the surface stained with antibodies that are always there where you have a population of cells that is able to rescue a mouse. And so you can use these to hypothesize that this stem cell marker is, in fact, marking the stem cells. And you can go and purify cells that are expressing a particular marker. And the way you do this is by using a really fantastic machine called the Fluorescence Activated Cell Sorter (FACS). I'll go through it very briefly in a moment. But what it is able to do is to purify cells that have got particular fluorescences. And they have these particular fluorescence spectra because of the way you have stained them for particular stem cell markers. And what you get out of your FACS machine is a population that is much more enriched for what I've called stem cells, but they're actually cells that are expressing this punitive stem cell marker. And then you can take these cells and say, well, have I really enriched for the stem cell, by asking how many cells you need to introduce into an irradiated mouse to rescue that mouse. And you can do a dilution assay where you put in one cell, ten cells, a hundred cells. Obviously, when you're down in the one cell range, you do your dilution assay, you get into a Poisson distribution, and you may not have exactly one cell. But you can do this statistically and you can figure out how many cells from your population it takes to rescue a mouse. OK? And the data to date tells us that one cell of the correctly purified population is sufficient to rescue an irradiated mouse. You have to do some tricks. You have to add some extra cells to this one cell. You cannot just put one cell into the mouse and rescue. You have to mix this one cell with some other cells that help it do its work. OK? But this is really an extraordinary assay that tells us we're very close to purifying a hematopoietic stem cell. What is a FACS machine? Very briefly. You can look at this later. Cells that are labeled with fluorescent antibodies are broken up into a stream of tiny droplets each of which will contain one cell. And the cells then pass in a stream through a laser which activates the fluorescence. There's a fluorescence detector that you can program to respond to a particular wavelength. And what happens is that when the detector finds that it has responded to a cell passing through it because of the fluorescence emitted, a charge is given to that cell, and that cell with a particular fluorescence moves down the stream through some deflecting plates where it will be deflected, according to its charge, into a collecting bin. And that way you can really get some very highly purified cell populations. You can purify about 300,000 cells an hour using the FACS machine. And we have a lot of them here at MIT and use them. Many laboratories use them for various things. A really cool machine. OK. But we need to move on. So we talked about some assays for stem cells. Talked about the potency of hematopoietic stem cells. I want to tell you something now about the control of stem cells and how a stem cell decides what to do. So if you think about organ maintenance, there is an implicit understanding that the organ knows how much it needs to be maintained. It needs to know how many cells are being lost, it needs to know when to make more cells, and it also needs to know if there has been some catastrophe. If there's been a liver, some kind of a resection of the liver, or if there has been skin wounding, OK, the organ needs to be able to respond. And the stem cells in an organ need to be able to respond to this catastrophe. And this is where this term niche comes in. Niche is one of these fields in the term, terms in the field. It's like the term progenitor. And niche is nothing that you haven't heard about before. What it refers to are the cells, as written up there, that surround the stem cell. And what I'm going to tell you is that the niche cells, the surrounding cells control, by induction, stem cell fate. And I'm also going to point out that there can be some input of the environment. So this is a cartoon that I drew for you. It's number six on your handout. It's six across on your handout. And I have here some stem cells that I've called quiescent. They are not dividing. Or they may be dividing just a little bit just to maintain themselves but not to give rise to any progenitors that will go on to differentiate. And these quiescent cells are maintained in this quiescent state, we believe, because they are surrounded by a group of cells that is maintaining them quiescent. What happens then is that these quiescent cells sometimes get a stimulus, some kind of environmental input, be it wounding, be it some kind of hormonal input from another part of the body that influences the surrounding cells and changes the surrounding cells such that they now change their patterns of gene expression and start secreting stuff, most likely, that changes the activity of the stem cells that they are surrounding. I've shown you arrows there indicating induction from the stem cells, from the surrounding cells to the stem cells. And what this inductive process does, just like in the early embryo is to activate the stem cells to divide and to form progenitor cells which will go on to do their thing and differentiate into various fates. And also, of course, the stem cells are renewed. So this notion of the surrounding cells telling the stem cells what to do is kind of new in the stem cell field. If one is a developmental biologist, it's one of these, OK, kind of observations because we know that cells signal to one another. And it's not surprising that stem cells are told what to do by the cell surrounding them. But there has been not very much information on this. And I want to give you one system where it's been very beautifully looked at and where the contribution of the surrounding cells has been looked at. And this system is the hair follicle. So your hairs come from a sheath of cells that starts off in the epidermis and moves deep down, extends deep down into the deeper layers of the skin called the dermis. And there is a shaft of cells of which these matrix cells down here are important because these matrix cells divide and they give rise to cells that will form the center of the shaft and secrete, or not secrete but synthesis proteins called keratins. The keratins are this stuff. And eventually the cell gets so full of keratin that it dies. And at the same time more cells are coming in the bottom here and this whole shaft, this whole hair shaft is pushed out of the hair follicle. OK? And so that is why your hair grows. It has been pushed out from the bottom. And all the stuff that we are so fond of is dead cells. It's actually protein. It's a very interesting polymer of various keratins. Now, what's very interesting here is that over the years researchers have shown that the hair, well, let me back track for a moment. You know your hair falls out, right? You brush your hair and your hair falls out. OK? Ergo, it must be replenished somehow. And over the years it has been shown that these bulge cells, or cells in a little bulge, which was a rather insignificant little bulge but it's there, sitting on the side of the hair shaft is actually the source of the stem cells for the hair. And another region, I'm going to tell you about in a moment, is down here. It's labeled DP. Bear it in mind as I tell you something and then we'll come back to it. So let me first show you some data that tells us that the bulge cells are the cells that contain or that include the stem cells for making hair. One can do an experiment where one takes these bulge cells and does essentially a repopulation assay, just as in the case of the bone marrow transplant. And one can do this into a strain of mice called nude. So nude mice have got multiple issues. But one of their issues is that they have no fur. OK? And one can take bulge cells from a normal mouse and transplant them into a nude mouse. Here's the control transplant, and you can see there's no fur. And here is a transplant of bulge cells from a normal mouse. And here you see this tuft of fur growing out on the back of the nude mouse. And, in fact, some very cool assays have been done where these transplanted cells are labeled green. And you can look at these tufts of fur and see them growing fluorescently green. So you know that the new fur came from the transplanted cells. OK. So what about niche? Well, you know that your hair falls out. But, actually, there's a lot more to it than that. And it turns out there is a whole hair cycle of when the hair grows and when it doesn't grow. And it goes like this. There's a time called anagen which is a period of growth when these matrix cells down here are dividing and they are pushing out this hair and it's growing. After a while the cells down here stop dividing and the hair follicle doesn't grow anymore, or the hair shaft doesn't grow anymore. This is called catagen. And it's a time that's termed regression. It is when there is no more growth. And if the hair is going to fall out, it might fall out then, or it might stick around and the next step might take place. And this is a step called telogen which is a resting state. And then after that there is a new state, a new anagen where the hair will start growing again. And it may either be a new hair or it may be the one that's there that just grows longer. It depends on the part of the body. OK. Now, what I'm going to tell you is that a region called the dermal papilla induces stem cell activation at certain times during the hair cycle. So look at this. At anagen, during growth, the bulge and this dermal papilla, OK, this DP, it doesn't matter what the name is but just look at the red, are far apart from each other. At catagen they're still far apart, but look at what happens at telogen in this resting stage. The dermal papilla and the bulge are touching one another. And this is the phase, although it's called a resting phase, this is the phase when the hair follicle is actually getting ready for the next big spurt of cell division and differentiation that is going to make the hair longer or make a new one grow. And then, subsequently, the dermal papilla and the bulge stay close together, but eventually they will start pulling apart again. Well, it's been shown very elegantly that this dermal papilla signals to these bulge cells when they're touching one another and tells the bulge stem cells to activate and start making more hair or a new hair follicle. And the signaling is done through a pathway called the wnt signaling pathway. And I point this out because it uses a protein that should be familiar to you, which is our old friend beta-catenin that we talked about way back when as being important for dorsal-ventral patenting in the early embryo. And I point it out to you to make the point that proteins are used over and over again. Regulatory proteins can be used over and over again in the body. OK. So this is a very lovely example of what happens in a stem cell niche. So let's move on and let's ask how plastic adult stem cells are. OK. So here we have some issues. Well, the goal of the medical community is to get a stem cell that can repair every tissue. And it may be that every adult tissue has a stem cell population in it, but these can be difficult to isolate. It's very difficult to isolate these hair stem cells. You can get enough to get tufts of hair on another mouse, but it's hard to get enough of them to repair someone's bald head for example. OK? So the Holy Grail of stem cell-ness is to try to find a cell that is a multi-potent adult progenitor. OK? And this has been the real goal of the adult stem cell field. And the question that has been posed is, is there a multi-potent, a highly plastic, multi-potent also termed plastic, adult stem cell? And if there was then in theory one could use it to repair all organs. So here again is our stem cell concept where some kind of stem cell goes on to give rise to a set of differentiated cells. Now, the set of differentiated cells forms what's called a lineage where all the cell types that come out of a single stem cell are related to one another because they come from a common heritage, from a common progenitor, from a common stem cell. And you can, for different lineages, make different trees of stem cells progenitors and differentiated progeny. And it has been hypothesized that there is some kind of interconvertibility between different stem cells in the adult, and that one stem cell for one lineage can be under the right circumstances converted to a stem cell for a different lineage. And that maybe there's even some kind of master stem cell floating around in the adult that can give rise to all other stem cells. Maybe there is some totipotent or pluripotent very, very highly potent adult stem cell that can give rise to all these other stem cell lineages and can, therefore, be used as this universal repair kit. And this has been assayed. And the prediction from this is that adult stem cells from one lineage, for example the hematopoietic lineage, can contribute to another lineage. For example, the muscle cell lineage. And this prediction has been tested in the following way. It's particularly been tested with hematopoietic stem cells. And the question has been posed can these hematopoietic stem cells contribute to other lineages? Here's a mouse expressing green hematopoietic stem cells, or making green hematopoietic stem cells because of expression of the protein GFP under an appropriate tissue-specific promoter. You can isolate bone marrow cells from the GFP expressing mouse. Transplant them into an irradiated mouse. You get rescue, as we've discussed, but that's not the point of the experiment. The point of the experiment, and this is the second to last slide on your handout. The point of the experiment is to ask not about the bone marrow cells in the rescued mouse, but to ask whether any of the other tissues in the mouse contain these green cells that would have arisen from hematopoietic stem cells. OK. So we're asking whether these hematopoietic stem cells can contribute to lineages other than the blood and the immune system. And this has been an enormous focus of research. And the answer initially appeared to be yes. And this was a cause of tremendous excitement. Here's one piece of data. This is a picture of some cells from a mouse that was rescued with green hematopoietic stem cells. And this is a picture of the muscle from the mice. The blue are nuclei and the green is the GFP that would have come from these transplanted hematopoietic stem cells. And you can see green cells also in the nervous system, nerve cells that appear to come from these hematopoietic stem cells. Well, it turns out alas that this is an artifact of the experiment. And, in fact, what happens is that these green hematopoietic stem cells rescue the mouse in the correct way. But then they also fuse together with other cells. They fuse with muscle cells and with nerve cells. The two cells actually join together. Their cell membranes mingle and you get a kind of hybrid cell that is a mixture of the hematopoietic cell and the regular muscle cell. And this whole notion of the adult hematopoietic stem cell contributing to muscle, to nerve, to various other lineages has completely fallen to disfavor in most fields, in most people's opinion. Although, there is still data, there is still much experimentation to do to really address this, both in the hematopoietic stem cell and other lineages. But no data currently supports that adult stem cells can interconvert one to another. And that leads us to the difficult position of asking, so where is this universal repair kit going to come from? And this is where we move into the field of, I'm going to just leave that now, of embryonic stem cells. So you know now that embryos have got many cells that are multipotent, pluripotent. And indeed the zygote, as we discussed, is totipotent. These cells can give rise to many, many different tissues because during embryogenesis that's the deal. That's what has to happen. And so it came to the attention of people long ago that embryonic stem cells might be a great source of stem cells that can be used as this universal repair kit. Now, I want to tell you, I'm not going to go through this, you can go and look on the PowerPoint, you can go and look on the PDF file on your website. I'm going to tell you about a type of cell, in the last few minutes, called the embryonic stem cell. And what you really need to know about this is it's almost totipotent. And the first slides, I'm not going to go through, show you that this embryonic stem cell type is almost totipotent. Now, what about these embryonic stem cells? They come from, in taking a very early embryo, at a stage called the inner cell mass stage -- -- when there is a little mass of cells in the developing embryo, that's going to give rise to the whole embryo. And taking this cell, removing these inner cell mass cells and putting them into a Petri dish with various culture media, what happens is that the cells from this early embryo divide and they make various clumps. And the clumps are descended from one particular cell. And each of these clumps of cells is called an embryonic stem cell line. Now, what's a cell line? A cell line is a population of cells that can grow continuously in culture. In other words, it self-renews. What's a stem cell line? A cell line, this is on your PDF file so you might want to sit and listen to this and then go and get this afterwards. OK? What is a stem cell line? A stem cell line is a cell line that has the potential to go on and differentiate into various different lineages. OK? And so from this embryo that has been put in a Petri dish and the cells allowed to grow you get out a number of stem cell lines. And the notion is that each of these stem cell lines, if treated with the correct factor that's called here a differentiation factor, can go on to give rise to different tissues. Heart muscle, pancreas, cartilage and so on. And the notion is that different ES cell lines will have different potencies. So some will be really good at making hearts, some will be really good at making muscle and so on. And it's not quite clear how one gets these different ES cell lines, but they exist from the mouse. And some of them exist from the human. And this is where we get into the other major issue of stem cell biology and the reason that there is so much legislature. Because in order to get these human stem cell lines you have to use a human embryo. To get human embryos you use methods of assisted reproductive technology. I sent you some notes on this and you can read this in the book. And the notion is that you remove eggs from a female, you make embryos in vitro, in a test-tube. And then when the embryos are balls of cells you harvest them, you kill them and you turn them into ES line. And the thing that is sticking people right now in the legislature and is something that is really worth thinking about is whether ethically it is OK, guys, it's a 11:54, so stay. Stay. OK. And the thing that is killing people, is really sticking people ethically is whether it's OK to take these human embryos and turn them into cell lines, because this does involve killing an embryo and this is an ethical issue. And I'm going to leave this here, and we'll come back to these ethical issues when we talk about cloning.