Flash and JavaScript are required for this feature.
Download the track from iTunes U or the Internet Archive.
Lecturer: Prof. Hazel Sive
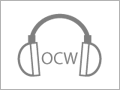
25: Nervous System 1
Related Resources
Biology terms (PDF)
So systems refer to, as in any case, something working together. In the case of biology, systems working together for a specific function. The tissues and the cells working together to a specific function. We're going to begin with a discussion of the nervous system. Let me write this on the board because you really need to know this definition. Many organs or tissues collectively giving one function. And we're going to begin with a discussion of the nervous system. The function of the nervous system is one of communication. But that single term communication really belies the complexity and the enormity of the nervous system. Could I please have a couple of letter carriers? Why don't you [do the other side? . So you can do each side. OK? All right. OK. So we're going to talk about the nervous system and its role in communicating. Communicating things coming from the outside in, communicating things that happen within your body. Within the nervous system there is one type of cell that is the most important cell with regard to communication. And the term, the name of that cell type is a, wait. I've got a new system here. A neuron. Very good. Excellent. Right. It's sort of your moment of fame. But you have to be careful, I might hand the microphone over for the rest of the lesson. OK. So the cell type that you need to know is the neuron. And the neuron is really a curious cell. In my opinion, it's one of the cells that is really one of the ancestral cell types. In fact, I think it might have been one of the first cell types because the thing that all cells really have in common, whether they live as single-celled organisms or as multicellular organisms, is a way of sensing what's around them. Even single-celled animals sense whether or not there are particular chemicals around them. I showed you in the morphogenesis section the dictyostelium, single-cells moving towards a cyclic AMP source. Those are single-cells sensing their environment. And I think ancestrally this kind of sensing of one's environment by a cell might have been the first function that really was encoded about the basic functions that allowed a cell to replicate in the first place. So if you look at all multicellular animals, this is taken from your book, all multicellular animals, you can find networks of neurons that serve as the wires for this communication network within an animal. The nervous system begins to develop very early during human development. By 19 days after fertilization you can pick out a region that is going to become the future brain. In studies in my own laboratory we've shown, using frogs and fish as models, that you can find cells that know, that are determined as nervous system cells when the embryo is still a ball of cells. So in human equivalent terms, when it's really less than two week's old, you can find cells that are expressing genes that are characteristic of the nervous system. So the nervous system starts to develop very early. Now, the cell type characteristic of the communication part of the nervous system is the neuron. And it has two interesting features. Its interesting features are called dendrites and axons. The dendrites and axons are both processes that project out from a cell body. Now, the cell body is where the nucleus lies, and these dendrites and axons are processes, some of which are extremely long. So this is a single cell. And some axons can be a meter in length. So there are axons that arise at the base of your spine, where they exit your spine, and they extend all the way down most of your legs. So they can be a meter or more long. That is a single-celled process. So that's very thin, OK? Not as thin as DNA but it's very thin. And it must be fairly robust. Usually axons are bundled together and tied together by other tissue to make them more robust. But they are very long. And neurons communicate by means of these very long processes connected one to another. And we'll talk more about that in a moment. Now, the way the nervous system is organized is actually rather peculiar and it isn't intuitively obvious that it would be organized in this way. Neurons, let me set this up, communicate, do a lot of their communication intracellularly, that is within one cell. And, actually, let me hold off on that until the next board. Within one cell, as opposed to having multiple little cells all next to one another that have conversations with one another. OK? So you'll have a neuron that extends an axon that could be as long as these tables. And that neuron will then communicate with another one next door in a kind of wiring diagram. You could imagine an alternate way of communication where you had a little cell sitting next door to another cell, another cell, another cell, another cell and so on. And you would get the same pathway, you'd get the same circuitry, but it would be an intercellular means of communication. And it's interesting to consider why that hasn't happened. It probably hasn't happened because it's faster, for reasons I'll explain in a bit, to communicate within the cell than between cells. So the neuron exploits for its communication its intracellular communication. Something that's present in all cells. And this is a potential difference across the plasma membrane. So all cells, as far as we know, have a potential difference across the membrane. And this is a number you probably ought to know. It's about 60 millivolts. Now, that doesn't sound that much, but if you extrapolate that into terms that we think about and think about the width of the plasma membrane which is just a few nanometers, that's actually a very large voltage difference. And if you do the calculations, you actually come to the conclusion that the potential difference across a cell membrane is about 100 fold higher than it is in high voltage power lines, OK, but it's very little. The actual numbers are very little, and so you don't realize that. But it's a very substantial potential difference. And what I'm going to tell you is that the neuron exploits this potential difference for intracellular communication. Now, all cells have got this potential difference. And in most cells, and there must be some reason all cells do, otherwise it wouldn't be maintained. But I must say for most cells it's not really understood why you have this potential difference across the membrane. OK. Moving on. There are lots of different shaped neurons. You can look at your book for a diagram. These are some actual pictures that are taken of silver stained neurons. Neurons take up silver dyes very effectively and show up as black. And you can see here is one with lots of processes. Here are some with much fewer processes. Here's one that is much, here are some that are much more tangled and so on. So there are many different shapes and forms of neurons. There are about 200 different recognizable neuronal cell types, and they're found in different parts of the nervous system and do different things. And as we move through these lectures, what I'm going to do is talk about today intracellular communication, next time I'll talk about communication between cells, and in the third lecture of the nervous system module I'll talk about how the wiring circuitry is set up in the organism. But today the focus is going to be communication within the neuron. All right. So I'm going to summarize that a little more because I want you to be with me. So some kind of input. The notion is that some kind of input impinges on the dendrites, the dendritic processes of a neuron. And these dendrites communicate this information often through the cell body, not always, to the axon of the same cell. And this axon then communicates to a dendrite of another adjacent cell. And that dendrite communicates with the axon and so on. So one can distinguish two different kinds of communication here. The first is the one that I have told you already, intracellular communication. And this is an electrical ionic movement. Well, this is an electrical communication. And it involves the movement of ions. And the second kind of communication is between one cell and another. This is intercellular across something called a synapse, or synapse, depending on your preference. And this is usually a chemical kind of communication, although in some instances it can be electrical. OK? And so, again, today's lecture we're going to talk about intracellular communication and on Wednesday we'll talk about this intercellular communication or synaptic communication. All right. So let me go through briefly on the board the notion of how a neuron sends a signal from its point of origin along the length of this very long axon, or usually fairly long axon or sometimes long axons. Axons can be very different lengths, but they generally are much longer than your regular somatic cell which is just about 10 microns in diameter. How is this intracellular communication affected? So what I'm going to do is to draw you part of a cell. We'll get to your diagrams in a moment, but I'm going to draw you part of a cell. So this is the axon, part of an axon. PM is the plasma membrane. And let me take just one piece of that plasma membrane and magnify it over here. So we can have out and we can have in. And the deal is this. The cell is set up, and we'll talk about how in a moment, such that it is more positively charged in the extracellular environment than it is in the intracellular environment. And that is stable. And it's stable at a particular potential that I've given you already, the 60 millivolts, which is called the resting potential. Now, along comes some kind of input, a sound, a touch, some food that you've eaten. And the neuron is told that there's an input. And what this does is in one portion of this axonal cell membrane, plasma membrane, it reverses the polarity transiently and very profoundly such that -- -- in this region, I'll just circle it. I think it's easier. In this region of the plasma membrane you have switched the polarity. This switching of polarity gives a change in potential difference. It almost reverses the potential difference completely. So whereas it's minus 60 millivolts in your resting potential inside to outside, it almost completely reverses to about plus 50 millivolts. And this reversal of polarity -- -- is given the name a depolarization or is a depolarization. And its neurobiology term, which you must know, is an action potential. All right. So this is very interesting, but what's even more interesting is what happens next. And so you should be asking at this point, well, how does that happen? And I'll tell you that in a bit. But what even more interesting is what happens next because that depolarization, that action potential then moves along the neuron. So if we draw a couple more, we actually only need to draw one more view of our cell membrane, after some period of time, and this is a millisecond timeframe here. After the millisecond timeframe, that initial depolarization reverses, and next door the membrane depolarizes. OK. So the signal then propagates along the neuron, along the axon in a particular direction. And you're going to ask now, well, how does it know which direction to propagate in? And I'll tell you that in a moment, too. Interestingly, the action potential is all or none. You either reverse the polarity completely or you don't. You don't get a little action potential or a big action potential. You get an action potential. OK? And that's important to know. So that is enough to start. And if you look at the handout I gave you today, if you don't have look at the one next door or come and zip down here and get one, that would be fine. I have in my first cartoon the outside of the cell and the inside of the cell. Now, I've shown these as completely positive and completely negative. That is a lie. They are not. OK? There is a potential difference. And so there's a charge distribution that's unequal, but it is certainly not completely positive and completely negative. That is for cartoon purposes only. So here's your resting potential and here's input from the outside of the cell. And what happens, to initiate this action potential, is that there is a small change in the membrane potential. It just changes a little bit such that it's now at minus 50 millivolts or so instead of minus 60 millivolts. And that is enough of a trigger to tell that membrane, and the membrane right next door, to depolarize and to give a full-blown action potential. So now that piece of the membrane has reversed its polarity. Positive charges have rushed into the cell. I'll talk about this more in the mechanism in the moment. Positive charges have rushed into the cell, or positive ions have rushed into the cell, and you've got your action potential. So the action potential is this reversal in membrane polarization, this depolarization. The action potential gives you an unequal charge distribution within the cell, so you've got these positive ions around. And they actually start defusing a little bit. Now, the axon is not a very good current conductor. Charges leak out of it. And very rather quickly and in an active way this charge in equality will be redistributed. But it does leak a little bit. It moves a little bit by diffusion. And, as it does so, it depolarizes the membrane next door just a tad to this threshold potential. And that will make this membrane next door amenable to an action potential and it will depolarize, and so will get an action potential next door. Now, I drew up here on the board that you get, in the first place where there was an action potential, the redistribution of charges again so that you get rid of the positive charges on the inside. And I've depicted this here. OK? So here are the charges from, this is all on your handout, guys, OK? These are the first, I believe, three figures on your handouts. So look at them and make notes, but you certainly don't need to draw these. OK. So here I've depicted the axis positive charges, the axis positive ions being moved back out of the neuron. And I've put these circles with a line through the middle which conventionally means no something or other. And what it means in this case is that this membrane that had just been part of an action potential is now no longer competent to elicit another action potential. There's some kind of inhibition that's been put on it so it cannot give another action potential. So it re-polarizes, but it cannot re-depolarize. And, again, there is about a millisecond timeframe window before it can depolarize again. And you can do the same thing. You can get the action potential moving up along the neuron. Here's the direction of propagation. And the direction of propagation is a result of diffusion, slight diffusion of the positive ions that are coming in during the depolarization that change the threshold, that change the membrane potential right next door to a threshold potential which allows the action potential to be triggered. And the propagation direction is unidirectional because the membrane that has just depolarized, that previously depolarized is no longer able to re-depolarize for some lag period. Yes? Maybe? Whatever? All right. We'll go with whatever category. OK. I always have to work at this. OK? I study this stuff and it takes me a while. So think about it. It's actually a very elegant strategy by the cell. You'll deal with this more on problem sets. All right. So let me move on. And let me move onto the question, actually, I'm not going to move onto this question. Yes. I'm going to move onto, all right. I will move onto this question. I'm going to tell you more next time that the action potential initiates at one particular place in the cell which is right at the place where the axon starts. And that is called the axon hillock. And we'll talk more about this next time when we talk about synapses. I do want to very briefly address the speed of propagation of the action potential. So it's been calculated that action potentials move at a speed such that you, if you ran the length of a football in one second, that is how fast the action potential is propagating down the neuron. OK? So you can do the calculation of how many meters per second it is. It's very, very rapid. OK? So you could get a nerve impulse in milliseconds going from one part of your body to another. It's actually interesting, though, it's not that rapid. And you've probably all had the sensation of touching a hot stove or something hot. And it takes you a moment before you actually realize that you've touched the hot stove. And then realize and you remove your finger. That lag, that moment of realization is a measure of your action potentials getting to your brain and then your brain saying, wow, or not even quite in your brain saying, ooh, I better move my finger. So that's actually a measure. There is a finite time that propagation takes. OK. Now, propagation of action potentials can be increased by increasing the diameter of a neuron. The fatter the diameter the greater the, the lower the surface area to volume ratio the more that current can move within the cell before being dissipated in various ways. And things like invertebrates, so the squid for example, has got axons which are a millimeter in diameter. They're called squid giant axons. They've very popular preparations to be used in neurobiological studies because they're so big. And they are very, they transmit an action potential very rapidly, or they propagate it very rapidly because they've got this high volume to surface area ratio. We do not do that to increase the speed of propagation. What we do instead is to wrap our axons in insulation. And the insulation we wrap our axons in is called myelin. It's secreted by cells called Schwann cells that are part of a system of support cells in the nervous system called the glia. And these myelin sheaths that wrap around the neuron do two things. Firstly, they prevent leakage of current as it moves along inside the axon. And so you get rapid propagation of current inside the axon. And, secondly, you get depolarization only at specific places where there is a break in this insulating myelin sheath. From your book, this is front your book. And from your book also I have taken this diagram where, it's actually hard to see here, but this is a break between the myelin sheath. It's called a Node of Ranvier. And here is where you get depolarization. The ions that cause the depolarization rapidly move along inside the axon to the next place where depolarization can take place. And that really increases the speed of propagation of a signal in our own neurons. All right. But, you might be asking, how is this potential difference set up and how is propagation occurring at the mechanistic level? I've thrown out charge movement, ion movement in a somewhat undirected way, but let's try to be more directed now and talk about what is actually happening. And the thing that's really important to know about is a set of proteins, actually, two sets of proteins. One are called ion channels and the other are called pumps. So the membrane is a wonderful thing. They insulate cells from one another. It allows the cells to function as a unit. And, indeed, it allows intracellular organelles to function as a unit. But there is an issue with the membrane in that it's insulating. And very little can get across it unless it's a hydrophobic something. So anything hydrophilic, including water, cannot cross the plasma membrane. And so you have to now devise, you've got this great invention of the membrane, but now you've got to devise a way to actually get things in and out of the cell. And this is where channels and pumps come in. So an ion channel is a protein, or usually a set of proteins that work together, that make a channel, a pore or a channel in the plasma membrane. There are a couple of different kinds of channels you should know about. All of them are selective. Selective means that they let some things through and don't let other things through. Some of them are open all the time. They also may be called leaky. I don't really like that term. I think open is a better term. And some of them have regulated opening. They're closed but they can be opened. And these are called gated channels. Another class of proteins that you need to know about are the pumps. Pumps are also proteins that sit in the plasma membrane. And they transport things across the plasma membrane, too, but unlike channels which are literally pores through the membrane and things can move through by diffusion, pumps use ATP to transport things across the cell membrane. And you can have things going into the cell, you can have things going out of the cell, and you can have things going both ways at kind of the same time. And ion channels are really key here in terms of setting up the membrane potential and propagating an action potential. This is a reconstruction of an x-ray crystalographic analysis of an ion channel. So what you can see is that there are nine, eight different proteins that are arrayed together to form this circle. And in the middle is a hole, literally, and that is the pore. And that is the pore through which the ion will move. And it's very interesting that the selectivity of an ion channel, well, how do you get a ion channel selected? Well, it hasn't been clear until this kind of analysis, which is fascinating. So here is a potassium channel down here. So this is something that lets potassium through, but it will not let sodium through, even though sodium is smaller than potassium, that sodium ions are smaller than potassium ions. So how does that work? Well, the way it seems to work is that the ions get through the pore if the pore doesn't know they're there or if the ion doesn't know it's going through a pore. So if the ion cannot distinguish whether it's interacting with water in solution or with the ion channel, it will be able to diffuse through the pore. If it can distinguish it thermodynamically it will not. So here's an example. Here is potassium interacting with water. Notice the spacing of the oxygens and the potassium. And here's sodium interacting with water. And you can see that since sodium is smaller the oxygen, the spacing of the oxygens and the sodium is closer, tighter. When potassium goes through the pore it turns out that there are a number of oxygen sticking out into the pore, and they interact with potassium in exactly the same disposition with exactly the same spacing as those water molecules in water. So this potassium ion cannot tell whether it's interacting with water or whether it's traveling through the pore. On the other hand, if the sodium ion were to get near the pore its charge interactions would not be the same as they are in water. It would only be able to interact with two of the oxygens and not the other two. And this is thermodynamically unfavorable, and it would not be able to go through the pore. So this is a very recent and very beautiful explanation of how pores can be selective. The ions go through one at a time in single file, and they can go through very rapidly because this is a diffusion driven process. OK. Now, what about these gated channels? I've shown here, from your book, a picture of an open channel and a gated channel that is open sometimes and closes at other times. The voltage gated sodium channel, we'll talk more about in a moment, is a particularly important channel. And this is a diagram that is, you know, it's a diagram but it will illustrate two points that I want to make. Firstly, you can find a state of a gated channel where it's closed and it won't let any ions go through. Upon a stimulus that channel will open and it will let the ions through. And then what seems to happen is that there's some kind of lash back where the channel says uh-oh, and there's some feedback that goes and closes the channel. But it's not in the same way that it was closed in the first place. And so I've called that an inhibition or closing or being refractory. And if you think about what I told you about propagation of information along a neuron, you'll be able to see why I am starting to tell you this. And then later on the system resets itself and is amenable to being used again. OK. I should point out this is an ion channel. This is a gated ion channel. The Nobel Prize a couple of years ago was given to Rod MacKinnon and a colleague whose name escapes me for getting the structure of these gated ion channels. And the notion is, I'll be an ion channel now. The notion is that in the membrane you're normally sitting something like this. OK? As an ion channel you get some kind of stimulus. The confirmation, and in the case of the neuron it's going to be an electrical stimulus, that slight threshold depolarization. And that is going to change the charge distribution on the proteins. Those proteins will undergo some kind of conformational something and they'll move like this and they'll open up the channel. OK? And we know a bit more than that, but the notion is exactly that. That changing the charge on the proteins of an ion channel, of a gated ion channel changes the confirmation of the protein and the channel opens up. All right. So I want to talk now, and I'll use the diagrams that I've given you, about how you generate the resting potential and how you generate the action potential in terms of the specific ions and the specific channels that are being used. All right. Channels that generate the resting potential, and actually I don't need to write this on the board because this is, these are going to be the last three diagrams, diagrams four and five and six on the handout that I gave you today. So how do you get this potential difference across a plasma membrane? Well, in all cells, not just neurons, in all cells it seems to be a function of something called a sodium-potassium ATPase. And I will write this. The sodium-potassium ATPase is a pump. OK? And its name kind of tells you that. The ATPase. It pumps sodium out of the cell and it pumps potassium into the cell. And you can look in your book for a bit more information about this. There is a fairly detailed understanding of the mechanism by which it does this. It's a very complex mechanism, but this gives you a gradient of sodium, or gives you unequal distribution of sodium and potassium ions across a plasma membrane. But, of course, that doesn't necessarily give you a membrane potential. That just gives you sodium on one side and potassium on the other side. So how do you get the membrane potential? Well, this is very interesting because what you do is use some channels that are open all the time. Now, what you've done with this sodium-potassium ATPase is to pump potassium in and sodium out. So you've got high potassium on the inside of the cell. If you open up the potassium channels, the potassium is going to diffuse down its concentration gradient and get out of the cell until there is some kind of charge pull and you get an equilibrium because it's being pulled back and pulled out and diffusing out with equal force. The sodium channels are closed, so sodium is trapped outside the cell. And these three things, the sodium being pumped out, the potassium pumped in, the open potassium channels and the fact that there are no open sodium channels gives you this unequal charge distribution across the membrane. Now, obviously there has to be some balance between this sodium-potassium ATPase action and the channels. Otherwise, you would not get a membrane potential. But there is. And the sodium-potassium ATPase pumps just enough that you maintain this membrane potential. OK. So that's your resting potential. How about your action potential? So your action potential involves another set of channels. And the channels it involves are these gates -- -- sodium channels. OK. So in the region, this is not animated, I'm going to tell you this. In the region of the membrane where there is an action potential, these gated sodium channels that are closed elsewhere open up and allow sodium to move down its concentration gradient into the cell. And they do it on a very mini scale, OK, over micron or submicron domains of the cell membrane. So you're getting this very little region of the membrane where these sodium channels are opening and they're voltage-dependent opening through this conformational change I belatedly demonstrated to you. At the same time there is a set of channels, which are gated potassium channels, and those are closed. There is a phase of repolarization that follows the action potential. And during this the gated potassium channels open up, potassium leaves the cell, and the gated sodium channels close up. And, as I mentioned, the ones in the vicinity of the action potential, or the ones that have just opened are now refractory for opening for another millisecond or so. So in the repolarization you use potassium channels, but a different set of potassium channels, a set of gated potassium channels. And you're also using the sodium-potassium ATPase during this time, because the sodium has to get out of the cell eventually. OK? And it will do so through the sodium-potassium ATPase exchange. All right. Here is a movie that will illustrate these different points. This is a diagram of an axon. I've got in a loop so we'll look at it a number of times. The membrane is shown in pink, and these boxes are the ion channels that are initially closed. And as the action potential moves along the axon they open up. So here it comes again. They're closed. And here they are opening sequentially to allow the action potential to propagate. Behind the action potential, take a look. You'll see that they remain closed for a while as it propagates. And then they eventually open up and reset themselves. And so this is a very beautiful example of an intracellular feedback loop, both a negative and positive feedback loop. And so here you're regulating. If you'd like to think of it in terms of regulating gene expression, you're kind of doing it at a very mini level. You're regulating gene activity, certainly, or the outcome, the products of gene activity. This is a diagram above. I haven't dwelled on this. You'll see this more in section of the depolarization, the action potential moving along. And you can depict that, as it's often done, by showing, by plotting change in potential against time. All right. Again, this is taken from your book. Here's a diagram of the threshold potential, the action potential and the repolarization. And I'm going to end off with a teaser for next lecture. OK? So that's what I want to say to you about the action potential. I'm going to end off with a teaser for the next lecture. These are two neurons. Each of the red dots, see the red dots? You probably cannot see them because there are so many. They kind of look like a red blur. But, in fact, they're each red dots. Each of those red dots is a connection between one of these two neurons with another neuron. It is a synapse. So we've talked about moving the signal through the neuron, but now we are at the point where the neuron, that axon needs to pass its signal onto the next neuron. And it's going to do it through the synapse, but it's not necessarily going to do it through one synapse per cell or one synapse per axon. It's going to do it through up to 1, 00 or even more perhaps connections from each axon to the next one. And what I'm going to talk to you about next time is how this is set up. We have a couple of minutes left so I will take you coming down here and asking me questions, and I'll finish the class now. Thank you.