Flash and JavaScript are required for this feature.
Download the track from iTunes U or the Internet Archive.
Lecturer: Prof. Tyler Jacks
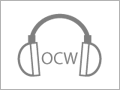
16: Cell Biology 1
Related Resources
Biology terms (PDF)
OK. I think we'll get started. We're moving into another phase of the course today talking about cell biology. There are going to be three lectures on cell biology covering a variety of topics. So we've been talking about cells in various contexts over the course of several lectures, actually, but more or less we've talked about them as static objects that carry out replication and transcription and translation and so on and so forth. But, in fact, as I'm sure you are aware, cells are highly dynamic structures which do many things at all times. And this is a movie from which shows the movement of cells. Cells will move randomly when you place them in a dish, but they'll also move in a directed fashion in response to factors in their environment that attract them or they'll move away from factors that repel them. So cell movement is an example of a dynamic process. Cells in the immune system, for example, are cells that do a lot of very critical movement to get to the site of a wound, to get to the site of an infection, and then to elicit the proper response to that infection. It's a late arriving crowd today. Another aspect of the dynamic nature of cells is the fact that they divide. And I've actually shown you this movie previously. From early embryogenesis through the formation of the fully formed organism and then throughout adult life, cells are receiving signals to divide at the appropriate times and to stop dividing when it's not longer necessary. This is a process of signal transduction, receipt of a signal which tells the cell it's time to do something, and then to process that signal in a way to leads to the proper cellular response. Maybe one of the most well understood or familiar examples of this process of signal transduction is the neuron depicted here in its actual state filled with a dye so you can see its various processes and diagramed here. Neurons, of course, are part of the information transfer in the nervous system where signals are received from these structures here called dendrites. That signal is then propagated through the body of the neuron through these long structures called axons and then released. The signal finally acts upon the structures at the very end of these nerve terminals leading to the release, for example, of neurotransmitters. These are impressive cells for a variety of reasons. And Professor Sive will actually give you some lectures which deal more specifically about signaling in the nervous system. One of the ways that they're impressive is their sheer length. You have neurons in your body that are more than a meter in length, and whales have neurons in their body that are tens of meters in length. So these are remarkable cells which carry out very specific functions. So basically what we want to talk about over the course of today's lecture, we'll get there eventually, we have to do some other stuff first, is the process by which cells respond to a stimulus. And then, much as a computer would do, to process that signal in very sophisticated ways, which I'll introduce to you this time and talk about in greater detail next time as well, to lead to a particular cellular response. And I've given you three examples of cellular responses in the movies and images this morning. For example, the cells might respond by moving in a particular directed fashion. They might divide because it's time for them to divide. Or they might, in response to this signal, release a neurotransmitter -- In the peripheral nervous system or in the brain. So what we want to understand is what does this really mean? What are the mechanisms that cells use to process signals? And you'll actually see analogies to integrated circuits as we go through some examples. Before we get there, we need to understand a little bit about how cells are built. Largely, but not entirely, the structure of a cell is related to its function. So neurons are an example. Their particular structures allow them to do the things that they do. Erythrocytes are another example. Small red blood cells, which are oxygen-carrying cells, are built the way they're built to do what they need to do. And largely, but not entirely, a cell's structure is determined by what proteins are expressed. And also where those proteins are found inside the cell. Where those proteins are localized inside the cell. So I want to spend a little bit of time talking about protein localization. So I want to spend a little bit of time talking about protein localization, how is it that proteins within your cells get to where they're going, in a sense know where they're supposed to go. And a critical tool for understanding this process of protein localization -- -- is fluorescence imaging. And so I think you're all aware of standard light microscopy where you pass white light through a sample, let's say a cell or a collection of cells, and based on the absorbance of the light through the different structures within those cells you're actually able to visualize what the cells look like. With fluorescence microscopy, what you do is shine UV light onto the cell, which brings energy to the cell and allows molecules that will fluoresce in response to that wavelength of UV to be visualized. So if you have a fluorescent signal within the cell, you can see where that signal is coming from using fluorescence microscopy or fluorescence imaging. So how is it that we get fluorescence from the particular structures, proteins or other things that we want to see inside the cell? Well, there are two general ways of doing that. The first is fluorescent antibodies. So let's imagine we have a protein of interest. We want to know where it is inside the cell. And we're able to make that protein, say, in bacteria. We can inject that protein into a bunny, that's a bunny, or into a mouse, that's a mouse. OK. These are the common organisms used for production of antibodies. We'll actually talk about how antibodies are made and how they recognize the things they recognize in subsequent lectures. But I think you all know that antibodies are made by the body to recognize foreign stuff. In this case, this protein that we've produced is foreign to the bunny or to the mouse. And so in response to that it makes an antibody, or a series of antibodies. These are proteins which have a particular structure that we often diagram like this. They have four chains, two big ones and two little ones. So these are antibodies. And importantly the antibodies are specific. That is they will bind to this protein but they won't bind to other proteins. So they specifically recognize this protein which is called the antigen in the context of antibodies. We can purify these antibodies and then chemically modify them to add a fluorochrome. A chemical moiety that will fluoresce in response to a particular UV wavelength of light. We can then apply those fluorescently labeled antibodies to cells that we've put onto a glass slide and actually poked holes in to allow the antibody to access the inside of the cell. And then we can visualize where the fluorescence comes from within the cell using fluorescence imaging microscopy. So, for example, if the protein of interest is in the nucleus, and we have labeled our antibody with a fluorochrome that fluoresces in the green wavelength, now when we visualize this cell by fluorescence microscopy the nucleus, if the protein is nuclear, will look green. OK? And this has been used in cell biology for a very long time, and it's still used today. It's a very powerful method for determining protein localization. This method is called immunofluorescence. Immuno for the antibody and fluorescence. And you can actually use multiple antibodies labeled with different colored fluorochromes and find multiple proteins in relation to one another. So that's one method. To an extent, this method has been replaced by a new method which relies on the fact that there are naturally fluorescent molecules. Naturally fluorescent proteins, I should say. You've actually all seen these. If you go to the beach, you might have encountered jelly fish which fluoresce. And they fluoresce because they make a protein which itself fluoresces when activated by the proper UV wavelength. These proteins have been isolated from these species, cloned into plasmids, and then used in recombinant DNA techniques, like I told you about before, to visualize where other proteins go inside the cell. And I'll give you some examples of that in a minute. But here's the jelly fish naturally. Here are these structures. The jelly fish actually makes a light which then causes these protein bundles to fluoresce green. The gene was cloned, as I said. It was given the name GFP for green fluorescence protein. And a cDNA copy of the mRNA is used in molecular biology all over the place to do an experiment like this. And I'll take you through an example of another one like this in a moment where you can actually fuse your gene of interest, gene X, cDNA corresponding to that gene with the GFP cDNA to make a fusion protein that has your gene of interest, plus GFP as one long polypeptide. And now this protein will fluoresce. And you'll be able to visualize it. And, again, I'll give you an example of how that's done in a moment. We've also used this, we, the field has used this to make organisms, other organisms which fluoresce. In my lab, for example, we've mad green mice which express the GFP protein in the cells of the skin of the mouse. So if you shine UV light on the mouse it turns green. It's kind of cool. And others have made pets that fluoresce. Surprising, but true, you can actually buy a fluorescent rabbit. I'm not sure who sells them, but they are available commercially. And I decided to show you a picture of one, of a rabbit called Alba the fluorescent bunny, which should be showing here. But Alba is actually a little bit shy so she doesn't always come. Alba the fluorescent bunny. Oh, here she comes. So this is actually a fluorescent rabbit which was made by introducing this GFP gene into the cells of the skin, such that it expressed in the cells of the skin of the rabbit. So it's a very powerful technique, but let me give you a few more examples of how it might be useful. I know. Everybody now wants one. So if you have a cDNA for GFP, and actually there are clever molecular engineers who have modified this gene GFP to produce fluorescent proteins that fluoresce in the red wavelength. So they're RFP, red fluorescent proteins. There are yellow fluorescent proteins. There are blue called scion CFP fluorescent proteins. So there's a whole kit, a whole coloring box, if you will, of different color fluorescent proteins that you can play with. You can then add that, in techniques that you are familiar with, to a cDNA of your gene of interest. So this is a cDNA of your gene of interest. If you now combine those using recombinant DNA techniques that we told you about in previous lectures, you could make a plasmid which has GFP fused directly in frame, to make a long open reading frame with your gene of interest. OK? And in that plasmid you would also include a promoter to allow the expression of this fusion. And it's also necessary to have, at the other end, a polyadenylation sequence, again, to insure proper expression of this fusion protein in the cells. And this would then be housed inside of a plasmid vector. OK? We could then transfect, introduce, using methods similar to transformation, but now not into bacteria. Instead into mammalian cells. We could introduce this plasmid into cells in culture so that in the nucleus of these cells that I've modified, I now have this new gene present which can express a green fusion protein with my gene of interest. Now, importantly, the goal of this experiment is to understand where the protein that's encoded by my gene of interest is localized. And the way that I'm going to do that is to follow where the green color goes. If it's a cytoplasmic protein the green will be in the cytoplasm. If it's a nuclear protein the green will be in the nucleus and so on. So if I now look at this cell by fluorescence microscopy, I will see the green signal in the nucleus. OK? It's a very, very powerful technique that allows us to follow the products of genes of interest wherever they might go inside of cells. And I just made a collection of images from the literature. Here you can see, actually, two different proteins being visualized simultaneously. The blue is actually a DNA stain. That shows you were the nuclei are. The green is one particular protein. And you can see that it's largely cytoplasmic. And the red is another protein rimming the plasma membrane of these cells. There are a whole bunch of cells here. You can see their shape by where the red color is. And the green is then marking the other protein which is largely cytoplasmic. In this example, similar to what I drew on the board, the fusion protein is in the nucleus. So the fusion protein is a nuclear protein. It drags the GFP with it into the nucleus. This is a structure called the endoplasmic reticulum, the ER. We'll come back to it later in the lecture. It's involved in the sorting of proteins to different parts of the cell. And here's an ER protein that's been fussed to GFP. So now the signal is coming from this structure inside the cell, the endoplasmic reticulum. And here is a protein which resides in the mitochondria, that energy-producing organelle inside the cell. You can drag GFP into the mitochondria to highlight those structures. Here's a red example, RFP fused to a cytoskeletal protein, so you now see these long filaments inside the cell now fluorescing red. And here's another kind of neat example. This is a protein that moves. It's normally present in the cytoplasm. As you can see here it's filling the cytoplasm of the cell and it's not in the nucleus which is labeled green. However, in response to a signal the protein moves. It goes from the cytoplasm into the nucleus. It might be, for example, a transcription factor, which is kept in the cytoplasm so that it won't turn on the expression of its target genes. But in response to the signal it now moves from the cytoplasm into the nucleus such that it can access the DNA and begin to turn on the expression of those genes. OK? So proteins can be followed dynamically. And you can actually do movies of these things over time. OK. So proteins can be moved from place to place. How do they get there? What is the mechanism by which proteins move from one compartment of the cell to another? How do they know where to go? There are two answers to this question. One is signals in the polypeptide. Signals within the polypeptide tell the protein where to go. These signals can be short, a few amino acids, four, five amino acids, they can be a little longer, 15 or 20 amino acids, but they mostly function as a molecular zip code. They can be read by the cell's protein sorting machinery. Like a post office reads a zip code, it knows to send certain letters to certain places. This machinery reads these signals and sends proteins to different places within the cell. There's also a separate and sometimes related mechanism -- -- known as post-translational modification. Here it's not a sequence within the protein amino acid sequence. It's not a signal within the amino acid sequence, but rather it's something that's added onto the protein after it's been made. That's why it's called post-translational. And, again, this can influence where the protein ends up inside the cell. So let me give you some examples of what I'm talking about here. With respect to signals that are contained within the polypeptide, proteins that are synthesized in the cytoplasm on ribosomes, this is a ribosome, this is a mRNA, 5 prime end, 3 prime end, will give rise, through the process of translation, to proteins. And when this process is completed, those proteins will be released into the cytoplasm of the cell. OK? Most translation occurs freely inside the cytoplasm of the cell. There are exceptions to that, as I'll show you in a moment. Most of it takes place freely in the cytoplasm of the cell, so when the process is done protein winds up in the cytoplasm. These proteins have two fates generally speaking. One is that they remain cytoplasmic. And I gave you some examples of cytoplasmic proteins earlier. Lots of the enzymes that are present inside your cells just hang out inside the cytoplasm and do their job. Other proteins are synthesized first in the cytoplasm will end up going to the nucleus, and these proteins are distinguishable by virtue of the fact that the ones that end up in the nucleus have a little tag on them. And this little tag has a name called the nuclear localization sequence. This is one of the short tags. It can be just four or five amino acids, usually positively charged. And inside the cytoplasm of this cell there are proteins which will recognize that little tag. Attached to it these proteins belong to a class known as importins. And what the importins do is drag the protein that has an NLS to the nucleus where they interact with a structure within the membrane of the nucleus known as the nuclear pore complex. And importin then drags the protein into the nucleus. It imports it into the nucleus. So nuclear proteins get into the nucleus by virtue of the fact that they have a nuclear localization sequence on them. OK? And you might imagine, and probably you will think hard about how you could visualize this process of nuclear importation, for example, in a problem set. So that's how cytoplasmic resident proteins are distinguished from nuclear proteins. What about the proteins that fully leave the cell? Many proteins that you make actually get secreted. They leave the cell entirely. Still other proteins wind up on the membrane itself. We're going to talk later in the lecture and next time about receptors that sit on the outside of your cell waiting for signals to be sent. Those proteins have to get there, and there's a special mechanism that insures that. So this applies to both secreted, as well as what we refer to as transmembrane proteins. And here the situation is a little bit different. In this case, when the ribosomes synthesize the very first few amino acids, the very first polypeptide chain, at the end, the end terminal end of these polypeptides, as they're emerging from the ribosome there is a longer, more like 15 or 20 amino acid sequence known as the signal sequence. If a protein has a signal sequence on its end you know it's going to end up as a secreted protein or a transmembrane protein. And when it emerges from the bottom of the ribosome, as depicted here, there's a specialized protein that will bind to it, it's actually a complex of proteins called the signal recognition particle. SRP has a very important function, actually two important functions. Firstly, when it binds to the ribosome, when it binds to the signal sequence like this it leads to translational arrest. It stops translation. It blocks the process of translation right at this point. OK? The next thing that happens is that it drags this complex of the ribosome with its little nascent polypeptide to a structure known as the endoplasmic reticulum. In the membrane of the endoplasmic reticulum. That was really loud. Is it that phone? I got to do this the other day in a class. Hello. Oh, they hung up. Last time I was teaching a class and the computer guy left his cell phone, and it rang. And the name of the person calling actually showed up on the screen, so I picked it up and I said Herb. And we actually talked for about five minutes and I told him I had to go and keep teaching a class. Anyway, inside the endoplasmic reticulum there's another receptor, not unlike the nuclear pore complex. This is called the SRP receptor. It binds to this thing SRP, signal recognition particle. And this then allows SRP to go away and allows translation to continue allowing the protein to be synthesized through this membrane and end up inside the endoplasmic reticulum. So the end result of this process is that the protein will now reside within this structure, this membrane-bound structure. Subsequently, the protein leaves this structure in a vesicle. It buds off the membrane, gets carried like cargo in a little pod coming off the mother ship here. It goes to another sorting structure called the Golgi apparatus. And it eventually goes to the plasma membrane where it fuses to the plasma membrane allowing this secreted protein to leave the cell. I realize that this is a little bit messy, but there are comparable pictures in your book. The point being that these proteins have a signal which drags them to the ER. There's then a sorting process that takes them out of the cell, takes them to the plasma membrane and then out of the cell. So that's how you deal with secreted proteins. How do you deal with proteins that end up in the membrane? If they don't get secreted they wind up resident in the plasma membrane. This happens by virtue of another signal. And I'm going to pick up this process, the beginning of the process is exactly the same. I'm going to pick up this process at a slightly later point where we have the SRP receptor bound by a ribosome. It's actually partway through the process of producing a polypeptide that has wound up inside the ER. For proteins that wind up ultimately in the membrane there's another sequence called a stop transfer sequence. And when a stop transfer sequence is hit and recognized by this complex it causes the ribosome to disassociate from the mRNA, or rather allows the synthesis to occur without continuous transfer through this complex. So the protein just gets built on this side of the membrane. And the result of that then is a protein that ends up halfway in and halfway out of the endoplasmic reticulum. If you then take this through the sort of sorting steps that I just outlined for secreted proteins, this protein will end up with part of it on the outside and part of it on the inside of the cell. OK? So that's how we deal with proteins that are transmembrane. And you'll actually see proteins that go through the membrane multiple times. That's because they have signal sequences and stop transfer sequence, signal sequences and stop transfer sequences that allow them to transition through the membrane more than once. Many of the proteins just once but other proteins multiple times. OK. So that's the way it's done in terms of signal recognition. I also mentioned that there is this class of posttranslational modification. Proteins get modified after they're made by translation. And the examples include proteolysis. The protein might actually get cleaved, cut. Half of it goes one place. The other half goes somewhere else. Phosphorylation. Addition of a phosphate can determine the localization of a protein. Glycosylation. Addition of sugar groups, carbohydrates onto the protein can determine where the protein goes. And finally lipid addition. Addition of a lipid molecule can determine where the protein goes. And I'll give you one example of that last one. A protein that we work on in my lab, the protein called RAS, which will actually come up in the next lecture. It's a signaling protein, which actually turns out to be very important in cancer. In its unmodified state the protein is cytoplasmic. However, it gets modified by an enzyme called farnesyltransferase. Which modifies the very end of the protein, the C terminal end of the protein, and it sticks onto it a lipid group, about a 20 carbon lipid group, which is very much like the fatty acid side chains in the plasma membrane. And, in fact, this is a very hydrophobic structure. And it ends up dragging the protein to the plasma membrane. Here's the plasma membrane. I'm drawing it now as a lipid bilayer, whereas I drew it as a single line previously. And that lipid tail, which has been stuck on the RAS protein, inserts into the lipid bilayer and localizes RAS then to the plasma membrane. OK? So here are examples of post-translational modifications that effect where the protein ends up going. So we can determine where the protein goes, that's very important. I also want to make the point before we move onto the next topic that protein-protein interactions are very important in determining function. These proteins that we've been talking about almost never, probably never do their functions by themselves. They interact with other proteins. And these are determined, again, by sequences that bind to one another between the two proteins. So this is actually a protein structure from a colleague here at MIT, Thomas Schwartz, solved by him. What you're going to look at hopefully, this is a ribbon diagram of two proteins, the one in blue and the one in pink. And you can see that these two proteins bind to each other based on amino acid residues at this interface between the two proteins. This ribbon diagram will be replaced by a space-filling model. This is what the protein really would like inside the cell. And here's that interface more clearly visualized with residues in light blue on one side, pink on the other that allows the two proteins to interact. So protein-protein interaction is extremely important, allowing proteins to interact either for a long time because they carry out some essential function together or very transiently. Protein-protein interactions can be long-lasting or very transient. And to just give you a visual reminder of that, the transient interaction, this I took a couple of years ago, Johnny Damon getting run into by Damian Jackson. A very transient but actually quite important interaction between these two baseball players. And then there can be longer lasting interactions. Here's Brad Pitt, well, it's not that long-lasting, I guess, but the point is that proteins do interact either very transiently or in a long-lasting way. Jennifer actually did the cutting out herself of this picture that she sent me. OK. Finally, proteins can change their shape. Proteins can change their shape in response to binding of other proteins or binding of cofactors that they interact with. And here's another movie from Thomas Schwartz. This is a signaling protein, much like the RAS protein actually. And you'll see that upon binding to a nucleotide here, the protein dramatically changes its shape. It actually unwinds a little bit and exposes a new structure for binding. And this is one of the critical ways that proteins participate in this signal transduction, signal processing function. They receive signals, change their shape, and this allows them to bind to other proteins or carry out other functions. OK. So I want to move now to the signaling introduction for the last 12 minutes or so. I started the day with this slide of cells moving around. They're receiving signals from one another or from the medium and they're responding to it. I also gave you examples of cells dividing or not dividing, cells differentiating, turning into one type of end-stage cell or another, changing their function. We'll talk later about cells that receive signals and kill other cells, cells that receive signals and commit suicide in a couple of more lectures. So cells will do very complicated things in receipt of various signals. And this general process is known as signal transduction. And basically it's the ability of cells to respond to things in their extracellular environment, stimuli in their extracellular environment. These could be physical stimuli, light, odorants, or they could be biological stimuli, peptides, proteins, hormones, which then get sent first from the outside of the cell and then to the inside of the cell leading to either short-term responses -- I'll give you an example of glucose secretion in response to a hormone epinephrine or adrenalin. This happens exclusively inside the cytoplasm of the cell. The signal is received, processed, and the result is the secretion of glucose. Other signals will make their way into the nucleus of the cell, and this might lead to changes in gene expression. New genes are turned. Others might be turned off. Changes in gene expression. And this might lead to a longer term response. And we'll give you an example of this in the next lecture. So how does this work? Well, it's different in every way. Each example is slightly different. I show you this slide to give you sort of a visual picture of what it's like, we think. Like one of these, what do they call them, Kineto-something or rather sculptures that you see at Logan Airport, for example, where a small perturbation at one end is propagated through a series of devices and leads to sounds and whistles and movements taking place within the structure. It's not unlike dominos. When you flip a domino at one end it can spread, increasing its effect because of the involvement of additional dominoes as it goes along a complex structure. We actually found, as we were looking at the Web for pictures of this movie of Mr. Domino, does anybody know what Mr. Domino is? I had no idea. It's a computer game, and they have a cool little animation which I thought I would show you. Here's Mr. Domino. And it's actually kind of relevant because he'll go and his signal is being transmitted along a linear path involving other types of molecules spreading out. Again, other types of molecules being involved, and so on and so forth. It's not a great example but it is kind of fun. OK. So before I give you a specific example, I want to give you some principles about signal transduction. And, again, the principles that are relevant here in biology are not dissimilar to signal propagation in electrical engineering, for example. Signal transduction in its simplest form means that something which takes some form, like a ligand binding to a receptor on the surface of the cell, changes its form inside the cell. It causes a change at the membrane to produce a different type of response inside the cell. Importantly, for almost all of these signal transduction pathways, what happens at the membrane is usually inadequate to change whatever process is to be changed inside the cell such that it is necessary for signal amplification to take place. And we'll give you examples of how signal amplification occurs in biology. But rather than making one square, in fact, you might make multiple squares. There's also the opportunity, and it's often the case for signal diversification, so that in response to the same signal, in fact, processed slightly different ways temporally or spatially with inside the cell you might make, sorry, let's make this a circle. You might make two different responses that coordinate the overall cellular response. There's the opportunity for what we call signal integration. So with respect to signal integration, you might imagine one signal which produces a particular response. A separate signal which produces a distinct response. But if you add the two together, that is if both signals are received simultaneously, you get some third response. So signal integration, again, not unlike electrical engineering. And finally signal modulation. So let's imagine again that we have, in some circumstances, a particular signal giving a particular response. If, in a different context with a different signal being received by the cell at the same time, instead of producing this response, there's an inhibitory effect so that nothing is produced. This signal inhibits the processing of this signal, so this is inhibitory. And you could imagine, and we know exists, the opposite such that in the presence of this signal plus now a distinct signal there's an augmentation of the response so that now you make much more of whatever you were making. So this is augmentation. So all of these things happen in the process of cellular signal transduction, cellular signal processing. At the beginning of this process, for at least the examples we're going to talk about, things happen at the plasma membrane. So here is the plasma membrane again. You can think of the plasma membrane as sort of the outside of your house with the antennas sticking up into the air waiting for signals to be received. Well, in biological circumstances, those antennas are polypeptide receptors. These are in this category. Or proteins that have transmembrane domains, get stuck in the membrane, and end up on the plasma membrane. These polypeptide receptors are sitting out there. There are often thousands of a given polypeptide receptor type on the outside of a cell. And these bind to ligands that fit inside them. There's complementarity between the shape of the ligand and the receptor such that you get specific binding of the ligand to the receptor. The consequences of binding of ligand to receptor we generally think of now as changing the shape of the receptor. Something that happens on the outside through binding of the ligand to the receptor is propagated through this polypeptide chain to a change in the structure at the inside. OK? It's as though I took this guy's head and I twisted it. Eventually his feet are going to move. The same thing here. If I make a change out here, I can propagate that change through the polypeptide chain and change the inside structure. So if you imagine a ligand which has a particular structure, I'm sorry a receptor that has a particular structure, when you add the ligand you now change the structure. And take a protein that might be inactive, this might have some enzymatic activity, from inactive to one that's active. Or another example, if I take this receptor and I bind the ligand, change its structure, I might now allow, based on this change in its structure, an interaction between this protein and some other signaling molecule, some other signaling protein. And, again, we know examples of all of those things. Usually, maybe always, the change that takes place at the plasma membrane, like the first domino that you flip, doesn't have enough energy associated with it to change the biological response. It's necessary to propagate that signal and to amplify that signal through various stages. This process of signal amplification is very important. And there are two general classes of signal amplification. One is the production of secondary signaling molecules called second messengers. And the example that we'll give you next time is the second messenger cyclic AMP -- -- which can be produced in large amounts following the binding of a ligand to a receptor, thereby amplifying the signal. And the other, which we'll give you as two examples next time, is enzymatic, very often kinase cascades. Enzymatic cascades where you turn on the activity of one enzyme, which turns on the activity of more other enzymes, which turn on the activity of still more other enzymes and so on. So, we'll talk about specifics related to these next time.