Flash and JavaScript are required for this feature.
Download the video from iTunes U or the Internet Archive.
Topics covered: Biochemistry 4
Instructors: Prof. Robert A. Weinberg
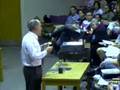
Lecture 5: Biochemistry 4
Good morning, class.
I just wanted to spend the first couple minutes clearing up three issues. None is a major conceptual issue, but we like to focus on details and get them right, get them correct here as well.
Firstly, I misdrew a reaction last time that described why RNA is alkali labile, i.e., if we have high pH we call that an alkali pH, or an alkaline pH, actually, to use the adjective. And we said that hydroxyl groups can cause the cleavage of the phosphodiester bonds of RNA but not DNA. And the way I described that happening is that the alkali group causes the formation of this five-membered ring right here, two carbons, two oxygens and a phosphate. And that resolves eventually to this where there's no longer any connection with the ribonucleoside monophosphate below. And I drew it like this, without an oxygen, and that's a no-no because, in fact, in truth, and as many of you picked up, this reverts to a two prime hydroxyl. So, please note there's a mistake there. There's also a couple other mistakes. For example, in the textbook it gives you the impression that when you polymerize nucleic acids you use a monophosphate to do so.
And, if you listened to my lecture last time, that doesn't make any sense, because you need to invest the energy of a triphosphate in order to create enough energy to generate enough energy for the polymerization. The textbook is incorrect there.
Textbooks are written by people, for better or worse, and as such, like everything else, they are a mortal and fallible. So, the truth of the matter is, when you're polymerizing DNA or RNA you need one of the four ribonucleoside or deoxyribonucleoside triphosphates in order to donate the energy that makes possible this polymerization.
And please note that is a mistake in the book. Recall, as I said last time, the fact that ATP is really the currency of energy in the cell, and that its energy is stored and coiled up in this pent up spring where the mutual electrostatic repulsion between the three negatively charged phosphates carries with it enormous potential energy.
And some of that potential energy can be realized, during the synthesis of polymerization of nucleic acids by cleaving this bond here. One can also generate potential energy by cleaving this bond here. This is the alpha, the beta and the gamma-phosphate. And cleavage of either can create substantial energy, which in turn can, as we'll indicate shortly, be invested in other reactions. The reaction of polymerization. A second point I'd like to make to you is the following, and you'd say it's kind of coincidence. The currency of energy in the cell is ATP, adenosine triphosphate, we see its structure here, and this happens to be one of the four precursors of the RNA.
So, the same molecule is used in these two different ostensively unrelated applications. One, to polymerize to make RNA where genetic information is stored and conveyed.
Or, alternatively it's used here in this context in order to serve as a currency for energy. High energy as ATP. ADP with a little lower energy. AMP monophosphate with even lower energy. And you might ask yourself, scratch your head and say why is the same molecule used for these two different things?
In fact, there are yet other applications of these ribonucleosides which also seem to be unrelated to the storage or the conveyance of genetic information. And it is believed, probably correctly, that the reason why the same molecule is used for these totally different applications is that early in the evolution of life on this planet there really were a rather small number of biological molecules that existed. Indeed, as we'll mention again later, it's probably the case that the first organisms didn't use DNA as genomes. It's an article of faith with us that one stores genetic information in DNA molecules.
And I implied that quite explicitly last time. But, the fact of the matter is, it's probably the case that the first organism, the first pre-cellular life forms used RNA as the genetic material, RNA to store things, replicating RNA via double-stranded RNA molecules as a way of archiving genetic information. And only later during the evolution of life on this planet, when that later was we can't tell, but it could have been a hundred or two hundred years later. Obviously, if we're talking about the origin of life as between 3. and 3.5 billion years ago, we can't really localize that in time very well, but only later was DNA assigned the job of storing, in a stable fashion, genetic information. And as a consequence, we come to realize as well yet another discovery, which is that all the catalysts that we're going to talk about today, the enzymes as we call them, almost all modern-day enzymes are proteins. And we talked about them briefly before. But over the last 15 years, 20 years there's been the discovery that certain RNA molecules also posses the ability to catalyze certain kinds of reactions. When I was taking biochemistry, if somebody would have told me that, I would have called the psychiatric ward because that was such an outlandish idea.
How can an RNA molecule catalyze a biochemical reaction?
It doesn't have all the side groups that one needs to create the catalytic sites for reactions. But we now realize, on the basis of research which actually led to a Nobel Prize being awarded about five years ago, that RNA molecules are able to catalyze certain kinds of reactions. And that begins to give us an insight into how life originated on this planet because RNA molecules may have stored genetic information, as I said before, RNA molecules, or their precursors like ATP, may have been their currency for storing high energy bonds, as is indicated here.
And RNA molecules may well have been the first enzymes to catalyze many of the reactions in the most primitive life forms that first existed on this planet. And, therefore, what I'm saying is that as life developed in the first hundred or two hundred million years, who knows how long it took, gradually DNA took over the job of storing information from RNA and gradually proteins took over the job of mediating catalysis, of acting as enzymes to taking over the job from RNA molecules. Today there are certain vestigial biochemical reactions which we believe are relics, echoes of the beginning of life on earth, which are still mediated by RNA catalysts. We think that they are throwbacks to these very early steps, maybe even in pre-cellular life form where RNA was delegated with the task of acting as a catalyst.
We're going to focus a lot today on the whole issue of biochemical reactions and the issue of energy. And this gets us into the realization that there really are two kinds of biochemical reactions.
Some of you may have learned this a long time ago.
Either exergonic reactions that release energy, that produce energy as they proceed, or conversely endergonic reactions which require an investment of energy in order to move forward.
So, here, obviously, if this is a high energy state and we're talking about the free energy of the system, which is one way to depict in thermodynamic language how much energy is in a molecule, if we go from a high energy state to a low energy state then we can draw this like this and we can realize that in order to conserve energy, the energy that was inherent in this molecule, the high potential energy is released as this ball or this molecule rolls down the hill. And, therefore, the reaction yields energy, it's exergonic. And, conversely, if we want this reaction to proceed, we need to invest free energy in order to make it happen. The free energy happens to be, more often than not, in the form of chemical bonds, i.e., energy that can be invested, for example, by taking advantage of the potential energy stored in these phosphodiester, in these phosphate-phosphate linkages indicated right here.
Here, by the way, is the space-filling model of ATP just for your information. That's the way it actually would look in life, and this is the way we actually draw it.
Now, having said that, if we look at the free energy profile of various biochemical changes then we can depict them, once again, in this very schematic way here.
And, by the way, free energy is called G, the Gibbs free energy after Josiah Gibbs who was a thermodynamic wiz in the 19th century at Yale in New Haven. And here what we see is that the change in free energy between the reactants and the products is given by delta G. So, by definition, we start out the reaction with reactants.
And we end up at the end of the reaction with products.
And, overall, if the reaction is exergonic and will proceed forward, it releases energy. And the net release of energy is indicated here by delta G. But, more often than not, biochemical reactions that are energetically favored, that are exergonic actually can't happen spontaneously.
They don't happen spontaneously because, for various reasons, they have to pass through an intermediate state.
Which actually represents a much higher free energy than the initial reactants posses. And this higher free energy, that they need to acquire in order to move over the hill and down into the valley, is called the energy of activation, the activation energy.
And, therefore, if I were to supply these reactants with energy, for instance, let's say I were to heat up these reactants and therefore give them a higher degree of thermal energy which they might be able to use to move up to this high energy state.
I supplied them with free energy by giving them heat.
Then they might be able to move up to here and then roll down the hill.
But in the absence of actually actively intervening and supplying them that energy, they'll remain right here, and they may remain right there for a million years, even though in principle, if they were to reach down here, they would be much happier in terms of reaching a much lower energy state. To state the obvious, all these kinds of reactions wish to reach the lowest energy state possible. But in real-time it can't happen if there is a high energy of activation. Now, what do enzymes do?
As always, I'm glad I asked that question. What they do is they lower the energy of activation. And this is in one sense obvious, and in one sense it's subtle, because enzymes have no affect on the free energy state of the reactants, they have no affect on the free energy of the products. All they do is to lower the hump, and they may lower it very substantially.
And because they lower it substantially, it might be that some of the reactants here may, just through a chance, acquisition of thermal energy, be able to move over the much lowered hump and move down into this state right here. Now, the actual difference in the Gibbs free energy is totally unaffected.
All that happens is that the enzyme, by lowering the energy of activation, make this possible in real-time. The fact is that ultimately, if one were to plot many kinds of reactions, many reactions, as is indicated here, have a very high activation energy, and therefore we look at it like this. But there could be other reactions which might have an activation energy that looks like this, almost nothing at all. And these reactions could happen spontaneously at room temperature in the absence of any intervention by an enzyme. For example, let's say we're talking about a carboxyl group which discharges a proton. We've talked about that already. Well, that reaction happens spontaneously at room temperature. It doesnt need an enzyme to make it happen. It can happen because there's essentially not energy of activation. But the great majority of biochemical reactions do have such an activation energy, and therefore do require a lowering like this in order to take place.
Now, let's imagine other versions of the energy profile of a reaction.
And keep in mind that what I'm showing here on the abscissa is just the course of the reaction. You could imagine I'm not really plotting time. I'm just talking about a situation where to the left the reaction hasn't happened and to the right it has happened. Can you see this over there? Then I won't write over there. All right. Let's see if this works.
Boy, here we are in the 21st century and we still haven't worked this out.
OK. Everybody can see this right here, right? OK.
So, look. Let's imagine we have a reaction that looks like this, a reaction profile that looks like this, where these two energies are actually equivalent. OK? I've tried to draw them on.
Well, they're not exactly, but they're pretty much on exactly the same level. And let's say we start out with a large number of molecules right over here. Now, if there were an enzyme around, the enzyme might lower the activation energy and, in so doing, make it possible for molecules to tunnel through this hill and move over here. The fact that when a molecule gets over here it has the same free energy as over there means that the catalyst may, in principle, also facilitate a back reaction.
What do I mean by a back reaction? I mean going in exactly the opposite direction. And so, once molecules over here are formed, the energy lowering affects of the enzyme may allow them to move in both directions. And, therefore, what we will have is ultimately the establishment of an equilibrium.
If these two energy states are equivalent then, I will tell you, 50% of the molecules end up here and 50% of the molecules end up here. And here we're beginning now to wrestle between two different independent concepts, the rate of the reaction and the equilibrium state of the reaction.
Note that the enzyme has no affect whatsoever on the equilibrium state.
These two are at equal free energies, the equilibrium state.
Whether the energy barrier is this high or whether it's this high is irrelevant. The fact is if the enzyme makes possible this motion back and forth, the ultimate equilibrium state will be 50% of the molecules here and 50% of the molecules there.
And, therefore, the enzyme really only affects the rate at which the reaction takes place. Will it happen in a microsecond or will it happen in a day or will it happen in a million years?
The enzyme has no affect whatsoever on the ultimate end product, which in this case is the equilibrium.
Of course, there is a simple mathematic formalism which relates the difference in free energies with the equilibrium.
Here we might have a situation where 80% of the molecules end up at equilibrium over here and 20% end up here. Or, we might end up as a state where 99. % of the molecules end up here and 0.
% of the molecules end up here. But that ultimate equilibrium state is no way influenced by the enzyme. They just make it happen in real-time. And, therefore, to repeat and echo a point I made last time, if most biochemical reactions are to occur in real-time, i.e., in the order of seconds or minutes, an enzyme has to be around to make sure they happen.
In the absence of such an enzyme of its intermediation, it just won't happen in real-time. Even though, in principle, it's energetically favored. So, let's just keep that very much in mind in the course of discussions that happen. And let's just begin now to look at an important energy-generating reaction in the cell which is called glycolysis. We already know the prefix glycol.
Glyco refers to sugar. And lysis, L-Y-S-I-S refers to the breakdown of a certain compound. I am not going to ask you, nor is anyone else in the room going to ask you to memorize this sequence of reactions. But I'd like you to look at it and see what take-home lessons we can distill out of that, what wisdom we can learn from looking at such a complex series of reactions. Perhaps, the first thing we can learn is that when we think about biochemical reactions, we don't think of them as happening in isolation. Here I'm talking about, for example, in this case I could be talking A plus B going to C plus D, and there might be a back reaction to reach equilibrium.
And we're just isolating that simple reaction from all others around it.
But in the real world in living cells most reactions are parts of very long pathways where each of these steps here indicates one of the others, a step in the pathway. What we're interested in here is how glucose, which I advertised two lectures ago as being an important energy source, is actually broken down.
How does the cell harvest the energy, which is inherent in glucose, in order to generate, among other things, ATP, which we've said repeatedly is the energy currency? ATP is used by hundreds of different biochemical reactions in order to make them happen.
These other biochemical reactions are endergonic, they require the investment of energy, and almost invariably, but not invariably, but almost invariably the cell will grab hold of an ATP molecule, break it down usually to AMP or ADP.
And then utilize the energy, which derives from breaking down ATP, it will invest that energy in an endergonic reaction, which in the otherwise would not happen. So, here we reach the idea that perhaps by investing energy in a reaction, the equilibrium is shifted. Because by investing energy, actually, the cell is able to lower the free energy state between these two.
And that makes it possible for their equilibrium to be much more favored.
Let's look at this glycolytic pathway. Glycolytic refers, obviously, to glycolysis. And here we start out with glucose.
We're drawing it out flat rather than the circular structure we talked about last time. And let's look at what happens here, again, not because anyone wants you to memorize this, but because some of the details are in themselves very illustrative.
The goal of this exercise is to create ATP for the cell, but the first step in the reaction is actually totally counterproductive. Look at the first thing that happens. The first thing that happens is that the cell invests an ATP molecule to make glucose-6-phosphate.
I've advertised the goal of this is to generate ATP from ADP, adenosine diphosphate. But the first thing here, this is an endergonic reaction in which the cell invests energy to create this molecule here. So, this doesn't make sense.
But ostensively it must make sense, at one level or another, because you and I, we're all here, and everybody in this room, at least this moment is metabolically active.
All right. So, we've got this molecule here, glucose-6-phosphate. And this can isomerize.
You see, here's glucose-6-phosphate, fructose-6-phosphate.
And, the fact of the matter is, there's no oxidation reduction reaction here. It's just an isomerization.
And this molecule and this molecule are virtually in the same free energy state. It happens to be the case that their profile will look very much like the one I drew you before. Their energy profile will look like this. And one needs an enzyme to lower it, but there's no energy that needs to be invested in converting one to the other because they're very similar molecules and therefore incomparable free energy states. Now look at the next step.
The next step is again another ostensively totally counterproductive way of generating energy. Because, once again, ATP, the gamma-phosphate, its energy is invested in creating a dephosphorylated hexose, fructose 1, 6-diphosphate where the numbers refer obviously to the identities of the carbon.
And now we have a dephosphorylated fructose molecule.
And so here you can actually see what the three-dimensional, what we would imagine closer to what the three-dimensional structures of these molecules look like. And we shouldn't focus this time on whether it's this or this. For all practical purposes, let's just focus on this pathway here. And here, for the first time, what now happens is that this hexose is broken down into two trioses, i.e., into two three carbon sugars.
And this is a slightly exergonic reaction.
It yields, it happens without the investment of energy.
And there's an enzyme, once again, that's required in order to catalyze it. But let's be really clear now.
Now we have to follow the fate of two molecules.
The first triose and the second triose. They have different names, but we're not going to focus on the names. One thing you notice about these trioses is that they're readily interconvertible.
Once again, we can image that we have a situation that looks like this. These are flipping back and forth.
And therefore, for all practical purposes from our point of view, these two are equivalent because they can be exchanged virtually instantaneously one with the other. Now, so far we've actually expended energy. We haven't harvested energy. But, keep in mind, the old economic dictum; you have to invest money to make money.
And that's what's going on here. The first thing that happens is we have an oxidation reaction. What's an oxidation reaction?
We want to strip some electrons, a pair of electrons off of this particular triose, the 3 carbon sugar.
And by stripping off a pair of electrons we donate the electrons from NAD+ to NADH. And here these structures are given in your book. But NADH, it turns out, is the electrons are pulled away from the triose and they're used to reduce NAD+ to NADH.
Keep in mind that in an oxidation reaction, one molecule that's being oxidized is deprived, is denied a pair of electrons.
The other molecule that's being reduced, in this case NAD, acquires a pair of electrons. And you can focus, if you want, about the charge of these molecules, one or the other. But, keep in mind, that in these oxidation reduction reactions, whether it's plus charged or minus charged is irrelevant. The real name of the game is the electrons. Forget about the protons, whether it has a plus charge or it's neutral. The real name of the game here is that two electrons are being used to reduce this molecule to this.
By the way, third mistake I forgot to tell you before, there's a double-bond in one of the pyrimidines in the book that doesn't make any sense. Whoever finds it gets a prize, but no one's figured out what the prize is yet. So, this double bond gets reduced. You see the difference between this and this over here. And this NADH, it turns out, is a high energy molecule. The street value of NADH is three ATPs, i.e., in the mitochondria NADH can be used to generate three ATPs, and that's worth something. So, NADH on its own is a high energy molecule. It can't be used for that many things, but it can be pulled into the mitochondria where it's converted to three ATPs.
So, we say, well, we're starting to make some money out of this investment because we've made, in fact, these NADHs.
See right here. Why do we say two NADHs?
Because there are two trioses we're working with, and each one of the trioses gives you an NADH. So, everything that's going on after this, starting from the top here, is now double because we're looking at the parallel behaviors of two identical three carbon sugars.
So, here we've so far generated, in principle, six ATPs.
How much did we invest already up to this point? Two.
We invested two but we harvested six. Already we're starting to make a little money because I told you the street value of an NADH is three ATPs on the black market. OK, so what happens next?
Next is another good thing. Each of the trioses, one can actually cause each of the trioses to generate an ATP molecule from an ADP. What happens here?
It turns out that this phosphate over here is actually in a pretty high energy state, in no small part because of electron negative-negative repulsion. And by stripping this phosphate off this high energy phosphate stripped off of this molecule here, whose name we will ignore, allows us to phosphorylate an ATP.
And since there are two trioses being converted, we're going to get two ATPs. So, in effect, now we're actually ahead. We started out investing two, we got six back from the NADHs, and we're getting two back here.
So, we've made two ATPs. This is a good thing. Keep in mind, ADP is lower energy, ATP is a high energy. Once again, we have an isomerization where these two molecules are at comparable states here and here, where the phosphate just jumps over to this state. And this hydrolyzes spontaneously and we get this molecule right over here, phosphoenolpyruvate at the end.
And, once again, we harvest two ATPs, one ATP from each of the trioses. And we end up, at the end of this reaction, with pyruvate. And you'll say this is terrific because we invested two ATPs, we harvested four, plus we got six from the NADHs, right? Two NADHs, each NADH gives us three each, so let's do the arithmetic. Let's do the balance sheet. We invested to begin with, with the one glucose, we invested two ATPs. That was early on. Then the return was first two NADHs, which I've told you equals six ATPs. Because an NADH is worth three ATPs.
This is so far good. And now subsequently we've made four ATPs so that the net yield looks pretty useful. Six plus four is ten minus two, a profit of eight ATPs from one glucose molecule.
This is terrific you may say, but there's a rub.
There's a catch. If glycolysis is occurring in the absence of oxygen, if that happens, then we have a problem here, because the only way that these NADHs can generate ATP is if there is oxygen around to take these electron pairs and use them to reduce an oxygen molecule. That is, by the way, part of the reason we breathe. Keep in mind that when you generate an NADH from an NAD molecule, you need to regenerate the NAD.
You can't just accumulate more and more NADHs. You need to regenerate the NAD. And, therefore, this NADH, with their electron pairs, the electron pairs have some to be disposed of. You have to regenerate NAD. You can't just make more and more and more of this. So, how do cells get rid of it?
Well, how they get rid of it is simple.
You take the electron pairs and you slap them onto oxygen, and that's really called combustion. And you get a lot of energy out of that. But what happens if all of this is occurring anaerobically?
Anaerobically means the reaction is occurring in the absence of oxygen.
Well, if you have a yeast that's growing 14 feet underground, this is happening anaerobically. If you have a yeast that's fermenting in a big keg to make wine or beer, it's also probably happening anaerobically. If you start running in a 100 yard sprint, or let's say you had to run a mile, then initially there's enough oxygen, there's a lot of oxygen around to allow you to get rid of these NADHs and dump the electrons that they have acquired onto the oxygen molecule. And that's fine.
That's worth a lot because, in effect, what you're doing is you're taking oxygen and hydrogen and you're combusting them together.
And that's great. But as you start running down the street, soon the oxygen supply to your muscles is going to run out, and soon a lot of the energy production in your muscles happens anaerobically. Why? Because you can't get oxygen quickly enough to your muscles, and therefore, for a period of time, you start feeling that burning sensation in your muscles because oxidation of NADH isn't happening. And these NADHs instead are regenerated by another way. How are they regenerated? The electron pairs of the NADHs, must be, are dumped back onto this molecule right here, pyruvate. They're not used to make ATP because they can't be used to make ATP because there's no oxygen around to accept the electron pairs that these NADHs have acquired.
And so, what happens with these valuable NADHs?
Under anaerobic conditions this doesn't happen.
These NADHs are used instead, their electrons are donated to our friend pyruvate here, these three carbon sugar.
And what happens, when they are donated back to the pyruvate, in order to regenerate NAD you need more NAD to pick up to use later in the reaction, to use over again in another reaction.
When you donate the electrons from NADH back onto pyruvate, what happens? You get lactic acid. Lactic acid is what makes your muscles burn when you're running very quickly and you can't get enough oxygen into them to begin to burn up the NADH.
So, instead of using NADH to generate ATP, it's diverted to make lactic acid. That's in one sense good because you regenerate NAD.
Why do you need to regenerate NAD? Because you need a lot of NAD around for the earlier steps in the reaction. Keep in mind, early in the reaction you need NAD here. If you don't regenerate it then glycolysis grinds to a halt. So, even though you make NADH and it's a good thing in principle, in practice it has to be recycled.
And if it's not recycled to make more new NAD to allow this step to happen then the whole glycolytic reaction will shut down and you're in a mess. However, sadly, in the absence of oxygen, the only way to recycle this is to dump these electrons not onto oxygen which is energy rich, it's dump them back onto pyruvic acid creating lactic acid.
So, you reduce this bond right here. So, you get CH, COH. This bond right here is reduced and you get lactic acid.
So, instead of a carbonyl bond here you have CH and COH right here, that's a reduction reaction. And now you're able to regenerate the NAD. And now you say that's a great thing. But, keep in mind, that now the entire glycolytic reaction, how much is our net profit now? Before I was gloating about the fact that we made eight ATPs, we netted eight ATPs out of this. What are we back down to now?
What's the whole net yield now? Well, the TAs can't answer.
It's two, because we invested two and we got out four.
And it's only two. Now, why is this so interesting?
Well, until about six hundred million years ago there wasn't that much oxygen in the atmosphere. And in the absence of oxygen this is almost the only reaction that could be used in order to generate energy. And about six hundred million years ago more and more oxygen from photosynthesis became dumped into the atmosphere.
And soon oxygen became available to organisms like our ancestors.
And then they could actually begin to recycle this NADH in a much more productive way. And as a consequence what happened, instead of having glycolysis yielding two, we could go up to this theoretical eight because the NADHs could now deposit their electrons on oxygen, which is much more profitable.
In fact, I've just told you now that in the absence of oxygen you can only make two ATPs. I will tell you, without providing it to you, that in the presence of oxygen you can make 34 ATPs.
And 34 is, we can agree, much better than two in the presence of oxygen. Higher life forms could not evolve until this much more effective way of generating energy became available. And, therefore, if our ancestors who lived longer than six hundred million years ago were very sluggish and they weren't very smart, the reason why they were sluggish and they weren't very smart is because they couldn't generate the energy that was required to efficiently drive metabolism.
The metabolism, anaerobic metabolism, i.
., occurring in the absence of energy, is extremely inefficient.
It just doesn't happen very well. Now, what actually happens if we have oxygen around? Well, what happens is something like this. We take the pyruvate, which is the product of glycolysis and which is this much more primitive pathway, and we dump it into the mitochondria. And now we generate through this cycle here, which I'm not asking you memorize, please, don't do that. We generate the reactions which go from here and get us up to this 34 ATP yield per glucose. And the essence of the citric acid cycle, which happens in the mitochondria, keep in mind that the mitochondria look like this.
Keep in mind that the mitochondrion are the decedents of bacteria which parasitized the cytoplasm of cells probably 1.5 billion years ago.
But if we now look at what happens in the mitochondrion, the pyruvate that we generated in the cytosol, in the soluble part of the cytoplasm is now pumped into the mitochondria, and there's a whole series of reactions that go on here, which takes this three-carbon sugar. The first thing that happens is that carbon is boiled off. Carbon dioxide, that's released.
Now we're down to a two carbon sugar. And then this two carbon sugar is added to a four carbon sugar and progressively oxidized.
And as it's oxidized what's spun off? Well, what's spun off is, for example, there's NADH which is spun off, there's ATP.
See, there's an NADH which is spun off. Here's an NADH that's spun off.
Here is a cousin of NADH. It's called FADH which, once again, generates a high energy molecule. Once again, the carbon molecules are oxidized, electrons are stripped away and used to create these high energy molecules, FADH and NADH.
By the way, FADH, a cousin of NADH, is only worth two ATPs on the open market. Whereas, NADH, as I've told you repeatedly, is worth three. And by the time we add up all of the NADHs that have been generated by this cycling and the carbon dioxides that are releases, at the end of this cycle here we start with two carbons, add it to four and we get a six carbon molecule.
We spew off some carbon dioxides here and go back to four carbon sugar. Add another two, go up to six carbons. Go around again, spin around the wheel. And each time we do that we generate a lot of NADHs, we generate a lot of FADHs, and we generate a lot of ATP. In all cases, these are highly profitable reactions simply because the NADHs and the FADHs can be used in the mitochondrion to generate ATP. So, let's look at the energy profile of the entire thing. Put it all together. This is where we started out at the beginning, and this is the end of glycolysis, OK? So, now we're adding up the energy profiles of the whole sequence of reactions that constituted glycolysis, which begins up here and ends right here because pyruvate, as you will recall, is the product of glycolysis, the first step. The Krebs Cycle happens, or sometimes it's called the Citric Acid Cycle. So, let's just get these words straight. Citric Acid Cycle because it happens to be one of the cycles, or it's sometimes called the Krebs Cycle after the person who really discovered it, Krebs.
The Krebs Cycle begins here. You see how the shading changes from pyruvate. And here we go all the way down there. And let's now look at what happens in terms of energy exchange.
Recall that early on we needed to invest ATPs to kick up the energy state up to here. We invested ATPs at this stage right here, and then we began to get some back.
We got these two NADHs, one NADH coming from each of the three carbon sugars. We got some more ATPs here and we got some more ATPs here, but these NADHs could not be used productively for generating ATP in the absence of oxygen, but in the presence of oxygen now we can begin to use these very profitably. Each of these makes three ATPs and each of these, obviously, makes ATPs. And then let's look at what happens in the mitochondrion. Keep in mind here's the borderline between the cytosol, the cytoplasm and the mitochondrion.
Here is where the oxygen is actually used and here we generate all these NADHs here, here and here, FADHs. And I keep saying, and it's still true, just in spite of the fact I keep saying it, that these NADHs can be converted to ATPs, and the ATPs can then be diffused, transmitted throughout the entire cell where they're then used invested in endergonic reactions.
Here we see all these NADHs. And look at the overall change in free energy. The initial steps in glycolysis didn't really take advantage. Glucose has inherent in it almost 680 kilocalories per mole of energy. It's pretty high up here. But by the time we get from here down to here, there's an enormous release of energy, it's harvested in the form of these molecules which are then reinvested.
In the absence of oxygen, this entire procedure can only go from here down to here. And a lot of this drop from six to seven is futile because we have to reinvest this NADH.
These cannot be used, actually, to generate more ATPs, as I've said repeatedly. So, this means in the end that we can generate an enormous amount of energy in the form of these coupled reactions. Having said that, let's actually look at what happens inside of the mitochondria.
Inside of the mitochondria there are actually different physical compartments. See the blue space there, the intermembrane space, the blue spaces there? The matrix is on the inside.
The intermembrane space is between the two, the inner and the outer membrane, and outside is the cytoplasm. The outer membrane, the inner membrane, in between it. So, look what happens, actually, in the mitochondrion. Those NADHs are used to pump protons from the inner space of the mitochondrion into the intermembrane space. I'm not showing you that happening.
But you'll have to take it on my word. So, protons pictured here are extracted from NADH and FADH, and they're used to pump protons out here. And, therefore, protons are moved from here to here.
Obviously, when you pump protons out the pH gets lower on the outside than it does on the inside, and because there's a gradient, there's a higher concentration of protons here than on the inside.
The protons begin to accumulate outside here in the intermembrane space. Are they in the cytoplasm? No. They're in the space between the inner and the outer membrane. You start to accumulate in this blue space lots of protons. And this pumping of protons into the space between the two membranes requires energy, and the energy comes from our friends NADH and FADH as it turns out. They are responsible for causing this accumulation of protons in the space between the inner and the outer membrane. So, now we get lots of protons out there. And what happens now, the protons like to flow back in because there is a higher concentration here as they are inside the space that's called the mitochondrial matrix, on the inside of the mitochondrion. So, what happens?
Here, yet another Nobel Prize winning discovery is the discovery of a very interesting molecule, or complex of proteins I should say, that looks in three-dimensions roughly like this.
And what this complex does is as the protons flow through the inner channel here, they're moving down an energy gradient.
They're going from a state of high concentration to a state of low concentration. What that does, that diffusional pressure actually yields energy.
And this complex right here harvests that energy in order to convert ADP into ATP. So, when I talk about NADH as being worth, each of them being worth three ATPs, what I'm really talking about is the fact that NADHs can be used to pump protons in the mitochondria outside here, and these protons can then be used, can then be pumped, can then flow in this way through this proton pump, which then uses ADP in the inner cavity of the mitochondria to create ATP. And here we get finally the conversion of ADP into ATP. We can realize, finally, this much promised benefit. And then these ATP molecules are exported from the mitochondria throughout the entire cell and used to drive many reactions. We've already encountered one important set of reactions, and those reactions are the polymerization of nucleic acids. Now, one final point I want to make is the following. We've just talked about metabolic, we've talked about the pathway of energy production in the cell.
And you might have had the illusion, for a brief instant, that those are all, that's the sum of all the biochemical reactions in the cell. But, in fact, if we plot out all the biochemical reactions in the cell, they're much more complicated. Here is the glycolytic pathway. You see it right down here where nothing is named? Here is the Krebs Cycle right here.
And we're not even talking about energy here. And as molecules move down this pathway from here to here to here to here, some of these molecules are diverted for other applications.
Not for energy production but for other applications.
And what happens out here, they are converted through a series of complex biochemical steps into other essential biological molecules. What do I mean by that?
If you give E. coli, a bacterium, you give it a simple carbon source like glucose and you give it phosphate and you give it a simple nitrogen source like ammonium acetate or something, E. coli can, from those simple atoms generate all the amino acids, can generate the purines and the pyrimidines, can generate all kinds of different complex biological molecules just from those simple building blocks. And so, the process of biosynthesis involves not only the creation of macromolecules, these steps of what are called intermediary metabolism are used to synthesize all the other biochemical entities that one needs to make a cell. They're used to synthesize purines and pyrimidines.
They're used to synthesize lipids, they're used to synthesize amino acids, and they're used to synthesize literally hundreds of other compounds. And when we see this chart like this, and nobody on the face of the planet has ever memorized this chart, each one of these steps, going from one molecule to the next, represents another biochemical reaction. And the vast majority of these biochemical reactions going from A to B to C to D.
Each one of these steps requires the intervention of an enzyme, a catalyst that is specialized for that particular step.
So, this begins to give you an appreciation of how many distinct biochemical steps one needs in a cell. The numbers probably to make a simple cell, you probably need about a thousand distinct biochemical reactions, each of one of which requires the involvement of an enzyme. And many of these steps, importantly, many of these biochemical steps are endergonic reactions. Where do they get the energy for driving these reactions forward if they're endergonic? ATP. So, the ATP from the energy generating furnace down here is the then spread throughout the cell to power all of these energy consuming reactions. Have a great weekend.
Free Downloads
Free Streaming
Video
- iTunes U (MP4 - 95MB)
- Internet Archive (MP4 - 95MB)
Audio
- iTunes U (MP3 - 12MB)
Caption
- English-US (SRT)