Flash and JavaScript are required for this feature.
Download the video from iTunes U or the Internet Archive.
Topics covered: Biochemistry VI
Instructor: Prof. Graham Walker
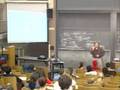
7: Biochemistry VI
Just very quickly, I mentioned to you the other day that article about Romney making a policy statement about embryonic stem cell research and said here were sort of examples of issues we were thinking about in this course that would come into your ordinary life. Here's on today's Globe there's, ìKennedy rips Romney over stem cell policy. This debate is continuing. It's on the front page of today's Boston Globe. We have two people neither of whom probably have the background in biology, that you guys are going to have by the time you finish this course, having to grapple with these very serious scientific issues that have all kinds of implications. Here's another thing. This was in yesterday's newspaper. This was on, I think, the second page of the Boston Globe. It's about a curator over at Harvard at The Museum of Comparative Zoology that got all these different birds, some of whom are extinct and something. And now the curator, instead of just having them there as a sort of collection and that's all it is, is getting DNA out of these specimens and working back and looking at gaining new insights from animals that aren't found on this planet anymore. And this was one was from, I didn't want to show you this on Valentine's Day particularly, but this is critical. I don't subscribe to The New York Times but for some reason one appeared on my porch on Saturday morning. I opened up the first page, ìRare and aggressive HIV reported in New York.î I'll be talking to you about the HIV-1 virus which is the virus that ends up destroying some of your major defender cells in the immune system. And then people die of AIDS which is sort of all the other stuff that then happens. And it was terribly scary when it first showed up. There was no treatment for it. It's like that in most of the Third World and people die if they get it. I lost a close friend to it after it first came up. Then they came up with these various cocktails of different inhibitors. And the idea was instead of just having a single drug target where it would be easy for the virus to mutate and, as I'll tell you, the HIV virus mutates like crazy. Instead they'd use multiple targets and that's why they do it. And sort of then the chances of it getting a mutation, you multiply all these low probabilities of getting a mutation and would hope it would never happen. It's happened. There in New York is a version of HIV-1 that is resistant to everything. If that spreads, which it probably will, it will be just like what happened with all the bacteria and antibiotics. It works for a little while, natural mutation and natural selection, something will happen, the organism or the virus will change so that it's no longer resistant. So, you know, for the moment, as always, you know, make sure you never expose yourself to AIDS. This would take us back just like to before any of these things were inhibited. And the same thing goes right now. There are strains of mycobacterium tuberculosis that are resistant to every known antibiotic. If you get tuberculosis with that thing it's like you were living 200 years ago. They cannot do anything for you. They'd put you in a sanatorium but you cannot be cured. This is worse. You know, this is awful. This one kills you. So just be careful. This is just the tip, but what always happens when you have antibiotics or drug treatments eventually natural selection mutation will probably produce a variant. And this looks like it is happening. I don't know, I hope not, but I'm afraid this won't be the end of this one. OK. So some of you, I've read some of the comments. I'm not terribly surprised that some of you think why is he telling me all this. God, there was a lot of work for tremendously confusing, there were all these chemical structures, I didn't really get it all. It seemed that somehow out of this the cell got to two molecules of ATP. Why are we wasting all the time? Why am I wasting all this time telling you about how to get two molecules of ATP? This is basically what we were doing. We were looking at glucose, which is C6H12O6, going through this process which pathway is of ten enzymatic steps. And what ends up coming out at the other end is two pyruvates. And the net yield of that is two ATP plus two molecules of this weird thing called NADH. Now, I think most of you, although a few of you still don't seem to have gotten it, the reason you need to make ATP out of ADP and inorganic phosphate is it stores energy. It's, you know, like having electricity in your house or batteries in your flashlight or in your iPod or whatever it is. You've got to have energy in order to do stuff. And cells need it. And the major sort of energy currency they use is ATP. And they put most of it by squeezing together a phosphate up to an ATP making this bond that's stable, unless there's an enzyme that'll break it open, but there's energy stored in it. And they'll drop downhill and you can use that energy to drive other reactions. So for life to happen it had to somehow figure out how to get energy in a form that it could use to do all the biosynthesis that's necessary for life to go on. NADH, I showed you the molecule, you got a structure. The cell makes some number of molecules of that. It's around. And what happened, it comes in, it helped some of these processes go. It ends up, it's sort of like a banking system for electrons. If it gets NADH it's got electrons stuck on it. There are only a limited number of molecules of NADH in a cell, so if you make all the NAD molecules into NADH then you have nowhere else to put electrons and everything comes to a grinding halt. Because I was talking about redox reactions. If you're taking electrons off somewhere they've got to go somewhere else. And the cell uses NADH as kind of almost a currency for passing electrons around in just the same way it uses ATP as a currency for carrying energy around from one form or another. Now, the problem was and why this was so important is this apparently, as I said, we're basically looking at an evolutionary fossil of some sort because every organism on earth uses, or virtually every organism on earth uses glycolysis. It's in our cytoplasm of our cells. It's in the cytoplasm of bacteria. It's in the cytoplasm of archaea. It apparently evolved so long ago that every form of life on earth, you know, depends on this, anything that can metabolize a sugar molecule pretty much. And so it looks complicated. It is. Maybe you could have designed, I'd like to tell you that it's two reactions and you make two ATPs and it's all simple. Life isn't often simple. It's often very complicated. It wasn't done by a team of design engineers in Building 10 designing it. It was done by something that happened with random selection. And when something finally worked apparently it got fixed in evolution even if it looks clunky. I think the other way to look at it is to say isn't that, I feel a sort of sense of wonder at this point, and granted I've had a lot of years working in the field, that something that complicated could work out and take this molecule of glucose and make it into two molecules of ATP that can then be used to allow the cell to do synthesize. As in I'll show you, there's a better way you could use this much more efficiently, 18 times more efficiently if we do respiration, which we'll talk about today, but that needs oxygen. And when life started 3.8 billion years ago or so there was no oxygen so it wasn't an option. The initial organisms had to make due with what they found and this is what developed. So ATP can go off and be used for lots of things. The problem, if there's no oxygen around, as I said there are a limited number of molecules of ATP. So to make that glycolysis go you're taking some electrons off and doing the oxidation steps, you're reducing NADH. And if you want that to go as a cycle you've got to somehow regenerate the NAD+ so you can go through another cycle and make more ATP. So there were two variants that I told you about. If you don't have oxygen around, one of these variants yields to lactate, and so the net yield of this is two ATP. Because what happens in this part is it uses up two molecules of NADH changing the two molecules of pyruvate to two molecules of lactate, so that gets rid of this, and the net yield of the whole thing is two molecules of ATP. The other way to do it is the way yeast does it. It makes two molecules of CO2 plus two molecules of ethanol. And I think when I wrote that on the board I showed you that there was acetaldehyde. We had two molecules of that going to two molecules of ethanol. And I think I forgot to write on the board that it used up two molecules of NADH because that's important. So that's what this whole thing is about. It achieves the same thing. And the net yield of this one is two ATPs as well. So I'm going to talk about photosynthesis at the latter part of the lecture, but if you maybe recall the initial sort of thing I called ìrelease oneî came up here about 3. billion years ago. Later on an improved version came along that started to generate oxygen. Now, it took billions of years for us to get to our present level of oxygen, but when oxygen became available in the atmosphere then there was a new option for making energy. And that was instead of taking this NADH and, remember, there are about 50 kcals per mole of energy in there, it's just being thrown away. It's not being used at all. But if there's oxygen around there's a new and better system, which you know as respiration, it's a word you've heard. You know we breathe oxygen. We need to breathe oxygen because we're using the oxygen to make energy. And I'll tell you again. You don't have to know all the details. But there's a biochemical cycle called a citric acid cycle. And it goes together with another process that's known as oxidative phosphorylation. And so this is if we have oxygen what we end up with at the end of the day instead of these products is six molecules of CO2 plus six molecules of water. But more importantly 36 molecules of ATP. So respiration is tremendously better at capturing energy from a glucose molecule. But this, I'll show you, is a later arriving development in evolution. It had to be because it required oxygen in the atmosphere. And so even though we only get two molecules out of ATP. We all do it. And the other thing it generates is pyruvate. And, as you'll see, this process takes those pyruvates. That's the starting material for this part over here. OK? I hope this is making a bit more sense as to why you've got to keep your eye on the big picture or all you're going to see is a whole lot of structures and chemical transformations that don't make any sense. This is all about making ATP and energy. And, as we'll see later on, photosynthesis. In cases it can be something to do with making reducing power for biosynthesis. So in order to understand this, though, I have to introduce you to another way of thinking about energy. Some of you still seem to be struggling with the idea that you can store energy in a chemical bond, but I think from the comments the majority of you have that. If you're having trouble, ask your TA or look in sections and stuff. But the insight to how this part came, even though this process, what underlies this was invented about roughly 3. billion years ago, scientists didn't begin to even get a glimmer of how this worked until about 1961 when there was a scientist named Peter Mitchell who got a Nobel Prize for the insight that he had. And what he recognized was there were sort of three forms of energy that are interconvertable. The energy of a chemical bond. And I keep telling you this, that ATP, if we hydrolyze that it goes to ATP plus inorganic phosphate. The delta G prime zero is about minus 7 kilocalories per mole, but under physiological conditions it works out that each ATP gets you about 12 kilocalories per mole because life doesn't happen under these standard conditions. So that's one form of energy we've been talking a lot about. There's another form of energy, which you probably know intuitively, and that is if you have a high concentration of some compound on a side of a sort of impermeable barrier and a low concentration on the other side, high concentration of sugar, low concentration of sugar. If you give the system a chance it will come to equilibrium. So the concentrated stuff will flow downhill until you're concentrated on both sides. There's energy that you could get out of that. So there's energy basically stored in a concentration gradient. There's another form which should probably be familiar certainly to some of you, and that's the idea you can store energy in an electrical gradient. If you have some kind of impermeable barrier and we have a lot of charges on one side and less charges on the other side then there's a polarity, there's an electrical gradient, and that's a form of stored energy. Now, it happens that a membrane is impermeable, as I've told you, to most things. So you can get a high concentration of something on one side and a low concentration on the other and controlled by a protein imbedded in it, whether those ever get a chance to come across. The same idea applies for an electrical gradient. And in particular of interest to biology are hydrogen ions. So if we have a situation like this where we have more hydrogen ions on one side of a membrane than another then we have an electrical gradient, we have a polarity. And so this is the membrane. And so all cells then have -- And the way it works is this is the outside of the cell and this is the inside of the cell, so more pluses on the outside, hydrogen ions on the outside than the inside. And it's about 70 millivolts. It may not sound that impressive, right? Another way to look at it, though, is the membrane is about three nanometers. So if you say, well, OK, what's the electrical gradient across that? It's about 200,000 volts per centimeter. And high tension lines are, you know, more like that per mile or something like that. So although it seems modest because the membranes, you've got have a very, very powerful electrical gradient in all cells. And so this is a form of energy. And I think this was proposed in 1961. Textbooks often say it was adopted in the early 70s. I went to a post-doc at Berkley in 1975 and people were still having arguments about whether this really was the way, was this really something that was used in nature? It is. And I think one of the most dramatic demonstrations that a proton gradient can be a source of energy comes from this sort of thing. I'd showed you how this bacteria, these are E. coli swimming around with these rotary motors that spin 10,000 to 100,000 RPM driving those flagella. I showed you the picture of the inner membrane of E. coli. And I mentioned it has a double membrane. This is sort of a protective layer. You'll see, a little bit later in the lecture, some double membranes again. And here's the motor with the big propeller, the flagella sticking out from it. And the way this thing, and I showed you, I guess, this picture and then this textbook diagram. What drives this motor are protons flowing from the outside of the cell through here, through the proteins in here, through channels in them. And that's what provides the torque for the motor. That's what drives it. It's not ATP or anything. It's protons on the outside and inside. And there's a very dramatic demonstration that's sort of like Friday night horror films, [if you will?], where people found a way of popping an E. coli open so that all of the cytoplasm ran out and then it would reseal. So what you've got is sort of an E. coli that is just a shell, just the membranes and the proteins imbedded in it, and it just sits there at neutral pH. If you now acidify the medium what's happened is you've created a proton gradient because there are now more protons on the outside. And this is just the same picture I showed you before. But what happens if you do that experiment is the bacteria start swimming. They don't have any insides or anything but you have created artificially a proton gradient and it drives the motor and they start swimming. It's sort of like ìdead man walkingî or something at a bacteria level. I think it's a really dramatic demonstration of how you can use the energy of a proton gradient to make energy. So the principle that underlies how respiration works and photosynthesis works is that you take advantage of this combination of concentration and electrical gradient. And it's known as the chemi -- -- osmotic hypothesis. Because here you have an electrical gradient because of the charges. You also have a concentration gradient because you've got more hydrogen ions on this side. So you cannot really separate them. They're kind of coupled. But the idea is that life uses this proton gradient in order to make energy and do some of these energy transactions. So here's the sort of principle of how it's done. You have a membrane like this, then we have a protein, and now we're not going to see all the alpha helices and beta sheets. It'll be one of those things we talked about that spans the membrane. The membrane, as you might guess from what you know about it, is impermeable to hydrogen ions. So what this protein does that's imbedded in the membrane, it's a proton pump. And if you provide it with energy in some form what it does is it takes a hydrogen ion that's on the inside and it makes it into a hydrogen ion on the outside. There's no chemical transformation of the proton. It's just gone from one side of the membrane to the other. It's almost like recharging a battery or something if you want to think about it perhaps in that kind of way. And then the second stage is once the proton gradient is established and you have now many more H+s on the outside than on the inside then there is another protein that lets the proton flow. The proton flows down to gradient and therefore is able to come back inside the cell. Now, if that's all there was to it we wouldn't have achieved anything. We would have wasted energy, pump something out, pump something back. But this molecule has an interesting property, and that is the ability of the proton to flow down the gradient obligatorily requires ATP plus inorganic phosphate. And as the proton comes down this energy gradient there's enough energy given off by that the cell is able to capture it and use that energy to synthesize a molecule of ATP. Got it? So you produce some energy like from the light in photosynthesis that we'll see in a minute, other ways of doing it, and then get it outside. Once you've got the gradient now you can make ATP. And, actually, one of the completely remarkable discoveries of structural biology, this is known as an ATP synthase. It's a protein. It's an enzyme. These are the kinds of things we've been talking about. This is also a protein. You see all the different things proteins do. So this ATP synthase, which uses this energy of the proton gradient to make ATP, its structure has been worked out and at a level, here's part of the crystal structure. You can probably see some alpha helices beta sheets. Here's a textbook diagram of it showing, it's upside down I'm showing, but here's a proton on the inside flowing across. And what's remarkable is it turns out that this ATP synthase is structurally related to the protein that's at the heart of that rotary motor that drives the flagella. And, in fact, remember, I think I showed you where you could stick the flagella to a cover slip and the bacteria twirled around so you could actually see they were rotating? So people did sort of the equivalent thing, they managed to stick this ATP synthase. And you couldn't see that the top part was turning, but they used some tricky stuff we'll talk about with antibodies and there's something to attach a long filamentous molecule. It's the polymer of proton called actin. That's the same stuff we find in our muscles. And it made it long enough. You could see that when this thing was working that it was twirling around. And so evolution took this same basic sort of piece of protein machinery. And in one case it used it to capture the energy of a proton gradient and make ATP. And in another case it used it to drive this propeller, if you will. And here's sort of a simple diagram. So as the proton flows kind of what happens is the inner part of this thing sort of goes click, click. And every time it does it synthesizes an ATP. And it sort of takes that energy to push together the ADP and the inorganic phosphate overcoming that activation energy and getting them close enough that you're able to get that bond. It's a truly remarkable thing. This is a somewhat hard concept to grasp, I understand, but if you can understand this endocrine variability between energy in the form of a combined concentration of electrical gradient and ATP, and nature goes back and back and forth, it's absolutely fundamental to life. If we didn't have this stuff going on we couldn't do it. You know, as I say with glycolysis, I wish it was simpler, but this is the way nature did it, this is the way we are, and [what I know about?] biology this is how it goes. And I wouldn't be doing it justice if I didn't tell you some of the details. You're MIT students. You should be able to, I hope, some of how the world actually works at this kind of level. OK. Now, with that kind of background, I think we can kind of -- Pretty quickly I can help you begin to see what happens here. Now, remember the problem up there with glycolysis? It started when there was no oxygen, and therefore it generated these NADHs. They weren't any good. You had to just get rid of them so you took the pyruvate, organisms learned how to put them to make lactate or ethanol and carbon dioxide, but if there's oxygen around then there's another possibility. And that is you can combine these molecules with oxygen. So if we take two NADH plus two hydrogen ions plus a molecule of oxygen what we get is two waters. And I think I can show you what's going on there kind of simply. What NADH was, we got a pair of electrons here and a hydrogen ion. Well, if we took that, what's that? I think you'd recognize it as a molecule of hydrogen gas. So if you take NADH and oxygen, what the cell is really essentially doing, it's taking hydrogen gas plus oxygen and it's giving two waters. So it's basically burning hydrogen. And I think most of you know what would happen if I had a mixture of hydrogen and oxygen up here and chucked a match at it or something. We'd have a massive explosion. And, in fact, that's why there are 50 kilocalories of energy released when that happens. And so on an energy sort of diagram, these free energy diagrams where we had the two NADH here plus the molecule of oxygen, and down here we have the two molecules of water, there is this. If that happened in one step it would be a huge amount of energy. No cell or organism on earth has figured out how to do that in one step. And I think some of the textbooks liken it to sort of say it would be like setting a stick of dynamite off in the cell. And this is where one of these things that seemed probably like a kind of uninteresting thermodynamic property, to some extent, becomes to be really important in understanding biology. And that is the fact that remember I said this drop in energy from reactants to products was a thermodynamic property? It didn't matter whether like you skied straight down the hill or you came down in a series of little things, you still got the same amount of energy release going from here down to there. So that's, in essence, what the cell does, is it takes this energy and it breaks it into little packages that it's able to manage in a chemical way. And so instead of coming down, and once it comes down in a series of steps, and every time it flows partway down hill it does something. And what it does is it passes two electrons to some kind of carrier. And then the two electrons flow downhill a little bit to another one and then to another one. And what happens when these electrons are flowing downhill, though, is that they drive that proton pump. So a proton pump takes the proton from being on the outside to the inside. And when the two electrons drop down to the next level another H+ goes from out to H+ in, again here. And if you understood what I said before, what the cell can now do is it can make three ATPs by using that ATP synthesis, and now taking advantage of those three pump proteins and making them into three ATPs. And then at the end of the day what happens then is these two electrons get together with two hydrogen ions plus I'll show it as a half of an oxygen here to give you water. And what's happening up here is, in essence, the molecule of sugar, C6H1206 is being burned with six molecules of water plus this to give you six molecules of C02 plus six molecules of ATP so that the cell is essentially achieving the same kind of thing by this process as if it had burned it in oxygen. There's that much potential energy released. And the total amount of this change in the d-prime zero is something out of the order of I think it's minus 686 kilocalories per mole. It's able to capture respiration. It captures about 60% of that energy as ATP. This process of fermentation, this is what these processes that happen in the absence of oxygen are known as fermentation. As you can see, there much less efficient. It gets more like 3. % of the energy captured as ATP. So you can see when oxygen arose in the environment what a huge boom it was to life because you could get a lot more energy for the same amount of starting material. There is one thing, though, that the cell has to do. In order to do this it has to do some more chemistry because it has to take those pyruvates and it has to somehow run them through this thing that I'm called the citric acid cycle and oxidative phosphorylation. Well, the oxidative phosphorylation is basically this chain I've diagramed for you here in a schematic way. And that's about the level you'll have to understand it. I mean physically it's going to be a bunch of proteins stuck in the membrane, and as the electrons get passed along they pump a proton as this pathway occurs. But the other thing the cells have to do is they have to take those two pyruvates and they have to burn them all the way down to C02 and water. And what's happening, if you remember that chain of oxidations, the carbons are being successfully oxidized all the way up to carbon dioxide. You cannot be anymore oxidized than that if you're carbon. What that must mean is something else is getting reduced. And what gets reduced, where the electrons go is NADHs. So once oxygen became available in the atmosphere then the name of the game was to take those two pyruvates and to somehow burn them all the way down to here, and therefore generate as much NADH as possible. And if you can make some ATPs along the way well and good. So there had to be a whole other set of chemical reactions that's every bit as complicated as glycolysis that emerged in nature, that carried out that job. And this time I'm not going to take you through all of the chemical structures. You can look at it in your textbook and stuff, but what I really want you to kind of take is to keep your eye on the number of carbons. If you look at the structure of pyruvate you'll see that it's three carbons, and it was this. Here's the keto group and an acidic group. So this is almost carbon dioxide. It's just one oxidative step away. So what happens in this cycle is that first the carbon dioxide is removed and this makes an acetate. In essence it's actually joined to something right here. And then this feeds into this thing called the citric acid cycle. And what it is, as I said, is it's a set of chemical reactions that are designed to take this pyruvate and burn it all the way to carbon dioxide and water, and to generate as many NADHs and ATPs as it can along the way. And, again, I wish it were simpler. It would be really nice if it just did it straight from acetate, but instead you'll see, if you look at the TCA cycle, the C4 compound. And this gets joined together to give us C6 compound. And then it oxidizes one of these carbons to give a carbon dioxide. Now you're down to the C5. It does it again, another molecule of C02, you're at C4. And then it takes this C4 carbon skeleton, puts it through some transformations that enables this cycle to go all over again. So basically the C4 thing just goes through the cycle. It's a carrier that takes this C2 right here, lets it be processed to give two molecules of C02. In that process it generates more NADH, a bit of ATP, and a kind of reduced carrier that you can think of for the moment largely equivalently to NADH. OK. Now, the entity in our cells that carries out this process of respiration -- Of respiration. So the citric acid cycle and the mitochondria is not actually in our cytoplasm, which is perhaps surprising, but all the enzymes for glycolysis are. So remember my simple diagram of the eukaryotic cell one the first day? We had the nucleus which is a membrane compartment that has the DNA inside it, and we'll have more to say about that in the next lecture of so, but then there were some organelles. And one of them I said was the mitochondrion. And I also said in the first lecture that there's pretty good evidence now that the mitochondrion arose by some earlier progenitor or ancestor of a eukaryotic cell capturing some kind of bacterium. It's actually one that's sort of kind of related to E. coli. It had double membranes. And the mitochondria actually still have some of their own DNA, but this is where all the energy, all the enzymes for the citric acid cycle -- -- and oxidative phosphorylation are found in the inside of the mitochondrion, instead of something that probably used to be a free-living bacterium. In contrast, the enzymes for glycolysis are in what's called the cytoplasm of the cell, which is sort of the main part of the insides of the cell. So you see even here this sort of evolutionary history that I kind of put out across the board. The enzymes for glycolysis arose so early in evolution they're in the cytoplasm of virtually every organism on earth. Eukaryotic cells manage to figure out how to get 18 times more energy for a molecule of sugar, but in order to do it they kind of had to cheat. They took a bacterium and figured out how to do it, put it inside our own cells, and then it's able to run now, it's become a part of our cell and it's where all the process takes place. And if you look at a structure of a mitochondrion it's actually got two membranes. You just saw a picture of that, an E. coli. And it's sort of involuted a little bit like this. And so this is an outer membrane of a mitochondrion. So I'm basically taking this and blowing it up. So this is a mitochondrion. And the in and the out, in this case there's an inner membrane. This is where the proton pump is located. This is where the ATP synthesis is located. And when a mitochondrion is working what it's doing is it's pumping hydrogen ions from its inside, which is more or less the equivalent of its cytoplasm, it's whatever used to be the cytoplasm of the original bacterium, outside into the space between the inner and outer membranes. And then it generates ATP by flowing back down. OK. So there are a couple of sort of things that affect your life that come out of this. One of them is understanding the ìfreshman fiveî or ìfreshman fifteenî or whatever it is. You come to college and all of a sudden you put on a lot of weight. Sometimes you can figure it out because you ate a lot of Ben & Jerry's or a lot of chocolate bars or something and didn't do as much exercise. Another one of you said, gee, now I understand why I'm not putting on any weight. I do water polo, and I guess I must be burning up everything I eat as energy. And you're right, but now I can show you at a molecular level what's happening. So what happens, your body, everything is regulated, and it can tell if it needs, cells in your body can tell if it needs energy. If it needs energy and you eat sugar it runs right through here and it makes ATP. But if you've eaten enough and the things that monitor your body say that you've got enough ATP then it doesn't keep this process going. Instead what it does is it stops it and says instead we're going to put something away for a rainy day, which you could see makes a lot of sense in evolution. You know, if things are good you just kill the mammoth and you can all eat well for a while or something. It would make sense for your body to be able to pack stuff away. So what it does is it intercepts the process at this level, at this C2 level. The pyruvate gets to here, and instead of being run through this in ATP this acetate or acetyl moiety which is a C2 gets run through a cycle of successive two carbon additions. And what comes out of that are fatty acids. It also takes three phosphor -- -- glyceraldehyde. You might remember that. That's one of the products stuck in the middle of that glycolytic pathway. So this is a three carbon compound. It makes it into glycerol which is a three carbon compound. And if you look back when we talked about lipids a couple of lectures ago you'll realize what happens if you combine fatty acids with glycerol then you've got fats. And, in essence, I mean that's what happens. We put on weight if we eat more than we're burning. Our bodies say, OK, I've got as much ATP as we need. I'm going to take some of that ATP and use it in this kind of way. Just a second here. I'm going to see -- So there's another thing that happens, a physiological thing that we experience. Well, we seem to be stuck at the moment. I won't worry about it. I've run some marathons. I don't know. A few of you may have. If not almost all of you have heard about ìhitting the wallî. And it happens generally around 21, 22, 23 miles. It depends on the condition you're doing. And physiologically I have experienced, this is amazing, I understand why they call it hitting the wall. You're running along and you think, boy, I'm tired but I'm doing OK. And in a space of a quarter of mile it's like you banged into a brick wall. And you can sort of keep yourself going but it's a profound physiological change. And what has happened at that point is you've run out of sugar to burn. Now, I've told you, you can take sugars and you can polymerize them into glycogen. That's one of those polymers with alpha 1,4 linkages. That's a sugar storage molecule. So if you start running, you carbo-load, you try and get as much sugar into glycogen as you possibly can. You start running the marathon. Your body starts taking that glycogen and breaking it down into sugars. It runs it through that process. What happens when you hit the wall as a human body is most human bodies are designed so that you run out of sugar. You just cannot carbo-load enough to do 26 miles. You can get through 20 or something there. And when you run out of that what happens then is your body can no longer burn sugar so it switched to burning fatty acids. And that's less efficient and you really feel it. If any of you have done long endurance things or triathlons or anything you've experienced that change. The final thing, just to close for the lecture for today, is one thing you can appreciate. Yeast is somebody that can do both of these things, right? It can either grow anaeorbically. And it makes C02 plus ethanol. Or it can grow aerobically. And this gives it two ATPs per glucose and this gives it 36 ATPs per glucose. So if you were a yeast, you would have to somehow regulate the rate of glycolysis depending on whether there was oxygen around or not. And it's very tricky and neat way it happens. There's an enzyme, that wouldn't surprise you, that has an active site for one of the key steps in the pathway where it's going from fructose-1-phosphate, fructose-6-phosphate to fructose-1, -diphosphate. That's one of the intermediate steps in glycolysis. It's the enzyme that turns out to be rate-limiting. You can control passage of something through a pathway by just making one step rate-limiting. So this has a place for the fructose 6 phosphate plus ATP to bind, and it catalyzes the formation of the fructose-1, -diphosphate. But that enzyme has to work at different rates depending on whether the cell is aerobic or anaerobic. So what it does is there's a second binding site over here. And if it binds ATP, this speeds up, excuse me, slows down the rate that's catalyzed over here. And that's what you'd expect. If it's got enough ATP it doesn't need to run glycolysis as fast. And it also binds AMP or ADP, and that speeds up the rate. So the yeast are able to monitor, to sort of have a control on how fast sugar flows through this glycolytic pathway. And what they're really doing is they're monitoring do I have enough ATP or not? And so if they're running with respiration, making lots of stuff, they don't need to do glycolysis as fast. If they're anaerobic they have to run it 18 times as fast to get the same amount of energy. OK? We'll begin the next lecture with photosynthesis and then we're going to get into a bunch of molecular biology. OK?